Micro-electromechanical system-based cryogenic and heating in situ transmission electron microscopy for investigating phase transitions and domain evolution in single-crystal BaTiO3
Abstract
Investigating phase transitions between ferroelectric states is crucial for understanding the nucleation, dynamics, and kinetics of domains, both before and after transformation. Here, we assess all phase transitions and domain evolutions in single-crystal BaTiO3 by implementing microelectromechanical systems (MEMS)-based in situ cryogenic (cryo-) and heating transmission electron microscopy (TEM) by continuously varying sample temperatures from -175 °C to 200 °C. Every possible phase-cubic, tetragonal, orthorhombic, and rhombohedral - was identified. An ultra-stable imaging condition was achieved with a mean drift speed of 1.52 nm/min, providing unique opportunities for atomic resolution in situ scanning TEM with a wide temperature range. Furthermore, domain nucleation and evolution across phase transitions were investigated using complementary dielectric measurements, optical microscopy, and a phenomenological model. This study underscores the effectiveness and utility of MEMS-based in situ cryo-/heating TEM in revealing phase transitions and domain structures in ferroelectric materials.
Keywords
INTRODUCTION
Improving tools and methodologies to characterize chemical and physical properties has been essential for studying the relationship between microstructure and macro behaviors, advancing various fields, including material and life sciences. Among all state-of-the-art technologies, in situ (scanning) transmission electron microscopy [(S)TEM] offers an irreplaceable advantage by providing direct observations of a sample’s response to external stimuli with high spatial resolution, ranging from the micrometer to even picometer scale.
Although the first electron microscope was built in 1932 by Knoll and Ruska[1], it was over 20 years later that the first cryogenic (cryo-) and heating TEM studies were reported, in 1954[2] and 1958[3], respectively. Subsequently, there was a burst of developments in in situ cryo- and heating TEM particularly in terms of the in situ TEM holder and following experimental applications from the 1960s to the 1980s[4]. In the early stages, there were two types of heating TEM holders: the ribbon-type and the furnace-type[4]. The former had advantages such as instant heating speed, but suffered from disadvantages such as inaccuracy in temperature control and image instability. To improve this, the calibration between the specimen temperature and the input power was essentially required, achieved by observing sharp changes in a material at known temperatures, such as phase transitions in a ferromagnetic material[5]. The latter offered uniform heating, excellent temperature stability, and precise temperature control. However, it had the drawback of longer heating times, typically taking 2-5 min to reach equilibrium temperatures[4]. Significant improvements to both types of heating holders were achieved through power-input temperature pre-calibration[6] and the thermocouple read-out method[7]. A substantial decrease in thermal drift was accomplished by thermally insulating the area between the hot stage and the microscope[8] and introducing a compensating opposite drift[9]. Combining a heating stage with an environmental cell enabled in situ observation of the gas-solid interactions inside the microscope from room temperature to over 1500 K[10-13], which was commercially available due to the efforts of Hashimoto et al. and Hiziya et al.[14-16].
Since the earliest applications of cryo-TEM to the present-day usage, the process has consistently required liquid coolant supplied directly from an external cryostat to the stage[17] or a coolant container installed within the microscope[18]. According to the different coolants, such as liquid nitrogen[19] and helium[17,18,20-25], the operating temperature could be extended from the room temperature to the liquid nitrogen temperature and below 5 K, respectively. In addition to extending the temperature ranges for cryo-TEM, versatility and reliability were also developed in the cooling holder’s design and function without sacrificing the spatial resolution[4,17,26,27]. These improvements include designing more convenient facilities for specimen exchange, enabling double-tilt capabilities of the stage between millimeter-sized pole pieces, maintaining mechanical stability despite turbulent and boiling coolant circulation, and ensuring thermal stability despite differing thermal expansions in the stage and specimen. Additionally, they involve eliminating contamination from residual vapor condensation inside the microscope during stage cooling, precisely controlling the stage temperature continuously before reaching the lowest temperature limit, and optimizing coolant consumption for greater efficiency. These advancements in cryo-TEM technologies laid the groundwork for later significant breakthroughs in the biological sciences[28], such as in studies of the frozen hydrated biological specimens[29-31], the vitrification technology[32,33] and viruses[34]. They also catalyzed progress in material sciences, enabling detailed studies of superconductors[22], solidified gases[18], and magnetic domains[35] from the 1960s to the 1980s. Over the subsequent decades, material scientists have pursued more stable working conditions in TEM in order to achieve higher resolution.
Over the last decade, the advancement of aberration-corrected (S)TEM has revolutionized atomic-scale imaging and spectroscopy, making these techniques widely and commonly used. This progress has reignited interest in in situ (S)TEM studies, leading to efforts to achieve native observations under various stimuli-such as heating, cooling, environmental (liquid/gaseous), mechanical, and biasing - especially at the atomic scale. Consequently, this high demand is driving the development of ultra-stable, versatile in situ TEM holders. For example, Gai et al.[36,37] focused on developing a heating holder combined with environmental conditions, based on earlier work[38]. They pushed the high-temperature limits to over 2000 °C[39], covering the temperature range required for the formation of ceramics, carbon nanotubes, and other materials. They demonstrated in situ characterizations of nanoparticle sintering with a resolution close to 1 Å[39]. Tai et al.[40] developed a prototype cryo-holder with an environmental cell in 2014, demonstrating the dynamic process of ice solidification with continuous temperature control between 220 K and 260 K. Later, Bell and Zandbergen[41] developed a side-entry JEOL-based cryo-holder in 2016, achieving less than 2 nm/min drift and sub-1 Å resolution in high-resolution TEM (HRTEM) imaging mode. In 2018, a Nion-based, microelectromechanical system (MEMS) chip-compatible, single-tilt version was reported, extending the operating temperature range from liquid nitrogen to ~1000 °C[42]. By 2020, Goodge
Despite many achievements in in situ cryo-TEM that have been reported, far less common are in situ multi-stimuli or so-called multi-modal TEM studies of the dynamics in ferroelectric materials, especially for covering the low to high temperature range in a single TEM operation. For instance, by applying in situ heating TEM, our previous work[56] demonstrated the dislocation-mediated domain nucleation and domain wall (DW) pinning in single crystal BaTiO3 (BTO), where the phase transition of room-temperature tetragonal (T) phase to high-temperature paraelectric phase was observed directly. Ignatans et al.[57,58] reported local hard and soft pinning effect of 180° DWs in bulk BTO by in situ biasing TEM at room temperature and the direct observation of individual Barkhausen pulses by in situ biasing TEM at 130 °C. O’Reilly et al.[59] demonstrated that in situ annealing of single-crystal BTO specimens at
MATERIALS AND METHODS
In this study, a commercially available [110]-oriented single-crystal BTO (GK EAST Optoelectronic Technologies, Inc., China) was used, which has a dimension of 4 mm3 × 4 mm3 × 8 mm3. [001]-oriented samples (defined in the pseudocubic coordinate system) with a dimension of 4 mm3 × 4 mm3 × 1 mm3
Dielectric permittivity measurements were obtained using an HP 4192A impedance analyzer (Hewlett Packard, USA) in the range from room temperature to 200 °C. These measurements were conducted under a 1 V root-mean-square (RMS) biasing field, utilizing a furnace (Nabertherm Inc., Germany) with a temperature ramp set at 1 °C/min. For measurements below room temperature, an impedance analyzer (Alpha-A, Novocontrol Technologies, Germany) equipped with a cryostat was used, also with a temperature ramp of 1 °C/min. Optical images were collected using an Axio Imager2 microscope (Zeiss, Oberkochen, Germany) equipped with a heating/cooling stage (HFS600E-PB4, Linkam Scientific Instruments, UK). Imaging was performed under differential interference contrast (DIC) and reflection modes. Bright-field (BF)-TEM experiments were conducted utilizing a Thermo Fisher Scientific Spectra 300 (Thermo Fisher Scientific, Inc., USA), operating at an acceleration voltage of 300 kV. The spot size 2 and the C2 condenser aperture of 150 µm were adopted. The objective aperture was 10 µm. The beam current was 3 nA. To acquire HAADF STEM images, the camera length was adopted as 115 mm. C2 aperture was 50 µm. The convergence semiangle was 21.5 mrad. The collection angle of HAADF STEM imaging was 50-200 mrad. The beam current was 50 pA. The multi-stimuli in situ TEM experiments, incorporating both cryo- and heating processes, were conducted using a double-tilt DENSsolutions Lightning Arctic holder[66] [Figure 1A]. BF-TEM images were acquired sequentially with a constant frame rate of 750 ms/frame. The temperature of the TEM specimen was increased from -175 °C to 200 °C at a rate of 10 °C/min, with pauses at -90 °C, 10 °C, 135 °C, and 175 °C, each lasting 3 min. After maintaining the specimen at 200 °C for more than 13 min, it was cooled down to -175 °C with the same rate, with pauses at 135 °C, 50 °C, 10 °C, and -90 °C, each lasting 3 min.
Figure 1. A: A DENSsolutions Lightning Arctic TEM holder for in situ biasing, heating, and cryo- experiments. The tip of the holder is internally connected to the metallic cooling braid that is immersed in liquid nitrogen, as depicted by the blue dashed line; B: thermo Fisher-compatible heating and biasing nano-chip for in situ TEM experiments. The scale bar is 2 mm; C: a representative SEM image of the FIB-prepared single-crystal BTO specimen on the nano-chip. A carbon protection layer (marked as FIB-C) was deposited on the top of BTO sample. The scale bar is 2 µm; D-F: finite element analysis simulations of the temperature distribution in the sample area during heating at a few set temperatures, namely -174 °C, -57 °C and 215 °C while the holder is being cooled. The scale bars are 100 µm. TEM: transmission electron microscopy; BTO: BaTiO3; FIB: focused ion beam; SEM:scanning electron microscope.
RESULTS AND DISCUSSION
A DENSsolutions Heating and Biasing chip (Thermo Fisher-compatible) was employed for the in situ TEM experiments, as featured in Figure 1B. This MEMS-based chip contains six electrical contacts, of which four are dedicated to control resistive heating and the remaining two are used to apply biasing stimuli [Figure 1B]. The tip of the holder is connected to a metal cooling braid via a cooling rod running through the holder. The cooling braid, attached to the back side of the holder, is immersed in a non-integrated liquid nitrogen dewar. Once the holder is cooled and stabilized, the heater on the nanochip can be activated to heat the TEM specimen locally to the desired temperature above the cryo-baseline, covering a temperature range from -175 ± 9 °C to the maximum temperature of 800 ± 40 °C or 1300 ± 65 °C, depending on the exact chip used, e.g., heating and biasing or heating only, respectively. The temperature calibration of the MEMS-based chip uses a linear relationship between the resistance of the heater and the temperature or by analyzing the infrared radiation produced by MEMS-based in situ systems[67-70]. More detailed characterization of the temperature calibration of the nano-chips in low and high temperature ranges will be reported elsewhere.
As shown in Figure 1C, a carbon protection layer (marked as FIB-C) was deposited on top of the BTO sample. This carbon protection layer does not significantly alter the domain dynamics due to its amorphous nature and minimal mechanical impact. The FIB-prepared TEM specimen, with dimensions of approximately 4 μm² × 3 μm² and a thickness of about 150 nm, was mounted on the window between two platinum pads solely through van der Waals forces[65], resulting in a free mechanical boundary condition for the ferroelectric TEM specimen. The BTO specimen was heated using a defined step-by-step temperature profile ramp. To validate the temperature distribution generated by the MEMS heater while the tip is being cooled, we carried out a finite element analysis using COMSOL Multiphysics[71]. As depicted in Figure 1D-F, the temperature distribution is homogeneous in the sample region at various temperatures. By using this experimental setup, we were able to observe phase transitions and domain features within a temperature range from -175 °C to 200 °C, covering the well-known phase transitions of BTO.
To validate the ultra-high stability of this in situ TEM holder at cryo-temperatures, capable of acquiring the atomic resolution HAADF STEM image, a sequential of ten frames of HAADF STEM images were obtained on this single-crystal BTO TEM specimen along the [001] zone axis. Each frame of the HAADF STEM image (2048 pixels × 2048 pixels) was captured within 1.29 s, resulting in 12.87 s in total for ten frames. The first frame is present in Figure 2A. The field of view of the HAADF STEM images is 18.37 nm × 18.37 nm. No obvious lattice distortion is observed due to the sample drift. To quantify the drift speed of the TEM specimen at cryo-temperature, the drift trajectory of a single Ba atomic column, representing the drift of the whole TEM specimen, is recorded by tracking the position of this single atomic column in sequential frames, as shown in Figure 2B. The starting point of this Ba atomic column is assigned as the origin. The direction and spread of position changes are shown by an arrow with gradient transparency. The average drift speed throughout the acquisition period is calculated to be 1.52 nm/min.
Figure 2. Drift analysis in the cryo-STEM experiment at -175 °C. A: A representative HAADF STEM image from a sequence of 10 frames. The scale bar is 5 nm; B: the drift trajectory of a sample, indicated by the arrow showing the direction and spread of position changes over time. The origin presents the starting position. STEM: scanning transmission electron microscopy; HAADF: high-angle annular dark-field.
To estimate the phase transition temperatures of BTO, we measured the bulk BTO sample with the same orientation during the heating process. Below -87 °C, our sample exhibited a Rhombohedral (R3m crystal structure) phase with a relative dielectric permittivity of 1600 [Figure 3A] and permissible eight spontaneous polarization vectors [Supplementary Figure 2]. The phase transition from the R phase to the O occurs at -87 °C, accompanied by a dielectric anomaly [Figure 3A]. The O phase has twelve spontaneous polarization vectors pointing toward the center of each edge of the unit cell [Supplementary Figure 2]. With increasing temperature, the O phase transforms into a T (P4mm crystal structure) phase with four spontaneous polarizations [Supplementary Figure 2] at around 12 °C, accompanied by a significant jump in the relative permittivity (△ε33/ε0 = 2000). Above this transition temperature, the permittivity decreased first and then increased up to the Curie temperature (TC = 132 °C). With further increasing the temperature, the permittivity decreased. Note that the permittivity values in the R, O and T phases measured in this work were higher than those reported in the mono-domain BTO crystal with the same orientation[72,73] suggesting that our BTO sample has muti-domain states in the ferroelectric phases[74,75]. The experimentally observed dielectric permittivity cannot be accurately calculated without further knowledge of the complex domain structure within the crystal.
Figure 3. A: Temperature dependence of relative dielectric permittivity (ε33/ε0) of the investigated single-crystal BTO sample measured at 1 kHz; B: calculated spherical map of bulk-free energy for C, T, O and R phases. The favored polarization vectors point toward regions with local energy minima (blue). The C phase represents a uniformly nonpolar state without distinct domains. R: Rhombohedral phases; O: orthorhombic; T: tetragonal; C: cubic; BTO: BaTiO3.
According to the Landau-Devonshire phenomenological model, the phase transition properties of single-domain BTO crystals can be described using a limited number of parameters. The bulk Landau free-energy density is expressed as an eighth-order polynomial expansion[76-78]:
Where αij, αijkl, αijklmn and αijklmnpq are the Landau coefficients. We assume that only αij are linearly dependent on temperature and the others are constants. The material parameters can be found in Supplementary Table 1. Figure 3B presents the calculated spherical maps of bulk-free energy for the different phases. In the C phase, the energy distribution is homogeneous, reflecting its symmetric and stable nature. Upon cooling from above the Curie temperature, the T phase emerges, characterized by four distinct regions (i.e., the four possible orientations of polarization vectors within the T crystal structure) of energy minima corresponding to the spontaneous polarization directions [Supplementary Figure 2]. Further cooling leads to the O phase, displaying twelve local minima that align with the polarization vectors toward the edges of the unit cell [Supplementary Figure 2]. Finally, the R phase exhibits eight regions of low energy[79], correlating with the spontaneous polarization directions [Supplementary Figure 2]. This comprehensive data illustrates the intricate relationship between temperature, dielectric permittivity, and phase transitions in BTO single crystals. The transitions from R to O, T, and finally to C phase highlight the complex structural changes that significantly influence the dielectric properties of the material. Understanding these transitions is crucial for manipulating and utilizing BTO in practical applications such as capacitors, sensors, and actuators, where precise control of dielectric properties is essential. The Landau-Devonshire model, coupled with the empirical data presented, offers a robust framework for predicting and explaining these phase transitions and their impact on material performance. The energy equivalence of various spontaneous polarization directions results in the formation of ferroelectric domain structures, which significantly influence the electrical properties of the material.
To monitor domain nucleation and evolution during the above-mentioned phase transitions, the in situ cryo- and heating TEM characterization is conducted on a single-crystal BTO specimen. The orientation of BTO specimen is further confirmed by the selected area electron diffraction (SAED) pattern taken at -160 °C, see Supplementary Figure 3. At -150 °C, both 71° and 180° DWs in the R phase are observed, which are not edge-on walls as shown in the BF-TEM image [Figure 4A and see schematics of these domain configurations in Supplementary Figure 4A]. Note that the 71° and 180° DWs have 0° and ~27° with respect to the reference [010] direction, respectively. Interestingly, a zigzag pattern composed of both 60° and 120° DWs is featured in the O phase [see the BF-TEM image obtained at -60 °C in Figure 4B and schematics in Supplementary Figure 4B]. The normal of these 60° DWs rotates with increasing temperature, rather than remaining fixed to any crystallographic axis[80], as seen in Supplementary Video 1. This zigzag to stripe pattern transition between O and T phases in BTO crystal was previously observed by piezoresponse force microscopy[75]. Additionally, edge-on 90° DWs are captured in the O phase, with the walls running along the [010] crystallographic axis. Note that both 120° and 90° DWs have walls parallel to the [010] direction, while the 120° DWs are not imaged edge-on. With further heating above the transition temperature of O phase to T phase, we document a-a and a-c type 90° ferroelastic DWs [see the BF-TEM image obtained at 50 °C in Figure 4C and Supplementary Figure 4C], with the boundaries running along the [010] and [110] crystallographic axes, respectively. The a-c type 90° DWs vanish and transform into a-a type 90° DWs once the temperature is higher than 60 °C [Figure 4D]. As the temperature approaches the TC, the a-c needle DWs disappear. Above TC, no distinct domain features are observed [see the BF-TEM image obtained at 200 °C in Figure 4E]. The stripe-like color contrasts in the image were typical bending contours in this case[81,82]. Detailed temperature profile is shown in Figure 4F and domain evolution during the complete in situ heating TEM process is provided in Supplementary Video 1.
Figure 4. A-E: Representative BF TEM images obtained along [001] zone axis of the BTO sample during in situ heating process. The scale bars are 500 nm; F: the plot shows temperature (T) vs. time, with points A-E highlighted at different temperatures, corresponding to BF-TEM images labeled A-E. T: Tetragonal; R: rhombohedral phases; O: orthorhombic; TEM: transmission electron microscopy; BTO: BaTiO3; BF: bright-field; DW: domain wall.
Figure 5 offers a comprehensive view of the domain evolutions observed through in situ cooling/cryo-TEM characterization across a range of temperatures, from the high-temperature C phase to the R phase at cryo-temperatures. Below the TC, the nucleation of needle-like domains and a1-a2 90° DWs are present, remaining stable below TC. This phenomenon is attributed to the large permittivity response of BTO, which arises from dielectric tensor anisotropy[82,83], where the permittivity of a domains significantly exceeds that of c domains (ɛa > ɛc). Specifically, at 75 °C, the BF-TEM image [Figure 5B] reveals the edge-on a1-a2 90° domains. As the temperature decreases, these 90° a-a type DWs transform into a-c 90° lamellar DWs below a certain temperature, with the domain boundaries becoming almost parallel to the [010] direction. This increase in DW density and the reduction in domain size may contribute to the observed rise in permittivity prior to the T-to-O phase transition, as seen in Figure 3. To double check the evolutions of domain structures in single-crystal BTO, we capture optical images as the temperature decreases from 140 °C to 30 °C. At 140 °C, the material is in the C phase, with no distinct domain structures visible [Supplementary Figure 5]. As the temperature drops to between 131 °C and 126 °C, a1/a2 DWs projected along the <110>pc axis begin to appear, indicating the transition to the T phase. In the temperature range of 128 °C to 122 °C, both a1/a2 and a/c domains coexist. Further cooling to temperatures between
Figure 5. In situ cooling TEM characterization of all four phases of BTO. A-E: BF TEM images obtained along [001] zone axis of the BTO sample. The scale bars are 500 nm; F: the plot shows temperature (T) vs. time, with points A-E highlighted at different temperatures, corresponding to BF-TEM images labeled A-E. T: Tetragonal; R: rhombohedral phases; O: orthorhombic; TEM: transmission electron microscopy; BTO: BaTiO3; BF: bright-field; DW: domain wall.
In ferroelectric materials, spontaneous electric polarization is typically coupled with spontaneous mechanical strain, forming domains with uniform dipole and strain. While domain formation reduces elastic strain and depolarization fields, it introduces gradients of spontaneous strain and electrical charge between adjacent domains, thereby increasing the energy due to DWs. The equilibrium domain structure in ferroelectrics is such that it minimizes the total energy resulting from these effects[86,87]. To reduce the spontaneous strain gradient, DWs ideally form on planes where the spontaneous strain is identical for both domains in any direction within the plane. These are termed permissible walls. Additionally, ferroelectric DWs are usually charge-free, meaning the spontaneous polarization on either side results in no net electrical charge, thus forming neutral DWs. When two domains with different spontaneous polarizations meet, the polarization vector and strain tensor transition from one state [P(−∞), e(−∞)] to another state [P(+∞), e(+∞)]. In the transition region, DWs with specific orientations are formed. The normal vector of the DW, n = (x1, x2, x3) of the DWs must satisfy mechanical compatibility conditions[80,88,89]:
where 𝑒ij(+∞) and 𝑒ij(−∞) are the components of the spontaneous strain tensor in each domain, and 𝑥𝑚, 𝑥𝑛 are the geometric coordinates. DWs can be classified into ferroelastic and non-ferroelastic types based on their ferroelastic properties. Non-ferroelastic DWs, where the spontaneous polarization vectors on either side are antiparallel, are referred to as 180° DWs. These DWs satisfy the condition 𝑒ij(+∞) − 𝑒ij(−∞) = 0, thus 𝑁 = ∞. Ferroelastic DWs, where the spontaneous polarization vectors are not parallel, are known as non-180° DWs, with 𝑁 = 2. As a result, 12 typical types of neutral DWs that meet mechanical compatibility in BTO system, identified by the phase structure (R, O, T) and the angle between the spontaneous polarization vectors (180°, 120°, 109°, 90°, 71°, 60°).
Based on our MEMS-based in situ cryo-/heating TEM results, we summarized the observed orientations of these DWs in Table 1. Note that the O60 DW is a typical S-type DW, whose normal direction nO60 is determined by the material’s spontaneous strain tensor[80]. The detailed set of plausible neutral and mechanically compatible DW types is schematically displayed in Supplementary Figure 4. It is noted that we are not able to find all permissible DWs in BTO; for instance, 180° DWs are not visible in the T phase in our BTO lamellae. It should be noted that 180° DWs in the T or O phase do not have a well-defined direction as they usually exhibit a meandering morphology in experiments. This may be caused by the DWs projected along the <100> pc or <010> pc axis, but not visible on the (001) planes. Our observations provide a detailed understanding of the domain dynamics in BTO single crystals, emphasizing how temperature variations influence the formation and stability of different domain structures. The in situ TEM characterization allows for real-time monitoring of these changes, offering valuable insights into the fundamental mechanisms governing the ferroelectric and ferroelastic properties of BTO. This knowledge is crucial for optimizing the material’s performance in various applications[84,90], including capacitors, sensors, and actuators, where precise control over domain configurations and transitions can significantly enhance functionality.
Cartesian components of boundary conditions and domain-wall normal vectors for polarizations in adjacent domain states for the inspected DWs
Wall | P(−∞) | P(+∞) | DW normal vector |
T90 | (1, 0, 0) | (0, -1, 0) | ( |
T180 | (1, 0, 0) | (-1, 0, 0) | (0, |
O90 | ( | ( | (1, 0, 0) |
O180 | ( | ( | ( |
O60 | ( | (0, | 𝑛O60 |
O120 | ( | (0, | ( |
R180{ | ( | ( | ( |
R109 | ( | ( | (0, 0, 1) |
R71 | ( | ( | ( |
CONCLUSIONS
In this study, we demonstrated HAADF STEM imaging under ultra-stable conditions with an average drift speed of 1.52 nm/min at the cryo-temperature, providing unparalleled opportunities for atomic-resolution in situ STEM imaging over a wide temperature range. We employed in situ cooling and heating TEM to investigate the phase transitions and the evolutions of ferroelectric-ferroelastic domains in single-crystal BTO vs. temperature varied in a continuous manner. By cycling the sample through a wide temperature range from -175 °C to 200 °C in a single in situ TEM experiment, encompassing the Curie temperature and the transition temperatures between the T, O, and R phases, we were able to examine the intricate behavior of the domains and DWs. We confirmed that BTO exhibits distinct domain structures and DW configurations in different phases. Specifically, we observed a dense, metastable configuration of competing a-c and a-a domain variants visualized through TEM. These observations were further corroborated by in situ cooling optical microscopy studies. The formation and behavior of different DWs were identified for all mechanically compatible and electrically neutral domain-wall types throughout the entire temperature range of the ferroelectric phases. To the best of our knowledge, this is the first time such experimental techniques have been utilized in this context. This novel approach allowed us to capture real-time changes in domain structures as the material underwent various phase transitions. The detailed mapping of domain-wall dynamics and phase transitions may offer invaluable insights that can improve theoretical models and simulations, thereby contributing to a deeper understanding of the fundamental mechanisms governing ferroelectric behavior.
The demonstration of the capabilities of the presented MEMS-based in situ TEM holder represents a promising step toward studying ferroic phase transitions across a continuous cryo-temperature range. In the near-future findings that combining a wide temperature range together with electrical stimuli will be presented. Furthermore, this study paves the way for future experimental and theoretical investigations using in situ heating and cooling TEM techniques. The successful application of these techniques to BTO suggests that they could be similarly applied to other ferroelectric materials, potentially leading to new discoveries and advancements in the field. Future research could explore the effects of external electric fields, mechanical stresses, charge distribution, and defect structures such as oxygen vacancies[91] and dislocations[56,92,93] on domain behavior and phase transitions. Our study demonstrates the power and utility of MEMS-based in situ TEM methods for studying the dynamic behavior of ferroelectric materials. The comprehensive analysis of BTO provided by this work not only advances our understanding of its phase transitions and domain dynamics, but also sets the stage for further research and development in the field of functional materials such as ferroelectrics and superconductors.
DECLARATIONS
Acknowledgements
We thank Dr. Chen Li and Dr. Mauro Porcu for their kind assistance and access to the latest instrumentation at the Thermo Fisher Scientific Center for Electron Microscopy (NanoPort) in the Netherlands. Tianshu Jiang, Yevheniy Pivak, and Leopoldo Molina-Luna undertook the electron microscopy analysis, and in situ TEM method development. Gijs van der Gugten conducted the Finite Element Analysis simulations, and Junjie Li conducted the theoretical calculations of Landau free-energy density. Fangping Zhuo provided the BaTiO3 sample, obtained optical images, and contributed to the interpretation of the results. We thank Dr. Alexander Zintler for helpful discussions.
Authors’ contributions
The conception and design of the work: Jiang T, Zhuo F, Molina-Luna L
Provided material: Zhuo F
The acquisition and analysis of data: Jiang T, Zhuo F, Pivak Y, Li J, Ni F, Molina-Luna L
The interpretation of data: Jiang T, Zhuo F, Molina-Luna L
The writing and revising: Jiang T, Zhuo F, Molina-Luna L
Writing and reviewing the manuscript: Jiang T, Pivak Y, Ni F, van der Gugten G, Li J, Zhuo F, Molina-Luna L
Availability of data and materials
The data that support the findings of this study are available from the corresponding author upon reasonable request.
Financial support and sponsorship
This work was supported by the German Research Foundation (DFG) through project nos (414179371) and (530438323). Jiang T and Molina-Luna L acknowledge the European Research Council (ERC) under Grant Nos. (805359-FOXON), (957521-STARE) and (101088712-ELECTRON). Zhuo F acknowledges the seed fund provided by Matter and Materials at TU Darmstadt project no. (40101529).
Conflicts of interest
Yevheniy Pivak and Gijs van der Gugten are affiliated with DENSsolutions BV, while the other authors have declared that they have no conflicts of interest.
Ethical approval and consent to participate
Not applicable.
Consent for publication
Not applicable.
Copyright
© The Author(s) 2024.
Supplementary Materials
REFERENCES
2. Leisegang S, Ross R R. Versuch mit einer kuehlbaren objektpatrone. In: Ross R, editors. Proceedings of the third international congress on electron microscopy. Royal Society London; 1954. pp. 184-88. Available from:https://scholar.google.com/scholar?hl=zh-CN&as_sdt=0%2C5&q=Leisegang+S.+Versuch+mit+einer+Kuehlbaren+Objektpatrone.+In%3A+Ross+R%2C+editors.+The+third+International+Congress+on+Electron+Microscopy%3A+Proceedings+of+the+Third+International+Congress+on+Electron+Microscopy%3B+1954&btnG= [Last accessed on 8 Oct 2024].
3. Whelan MJ. A high temperature stage for the Elmiskop I. In: Bargmann W, Möllenstedt G, Niehrs H, Peters D, Ruska E, Wolpers C, editors. Verhandlungen. Berlin: Springer Berlin Heidelberg; 1960. p. 96-100.
4. Butler EP. In situ experiments in the transmission electron microscope. Rep Prog Phys 1979;42:833-95.
5. Martin CJ, Boyd JD. A method for calibrating a specimen-heating stage in the electron microscope. J Phys E: Sci Instrum 1973;6:21-2.
6. Clark JB. High-temperature, high-resolution metallography. Metallurgical society conference 1965,38:347. Available from: https://cir.nii.ac.jp/crid/1570291224290647040 [Last accessed on 8 Oct 2024].
7. Jouffrey B, Favard P. Microscopie électronique à haute tension 1975: textes des communications présentées au 4e congrès international, toulouse, 1-4 september 1975. Societe Francaise de Microscopie Electronique; 1976. p. 345. Available from:https://scholar.google.com/scholar?hl=zh-CN&as_sdt=0%2C5&q=Jouffrey+B%2C+Favard+P.+Microscopie+%C3%89lectronique+%C3%80+Haute+Tension+1975%3A+Textes+Des+Communications+Pr%C3%A9sent%C3%A9es+Au+4e+Congr%C3%A8s+International%2C+Toulouse%2C+1-4+September+1975.+Societe+Francaise+de+Microscopie+Electronique%3B+1976.+pp.+345&btnG= [Last accessed on 8 Oct 2024].
8. Swann PR, Humphreys CJ, Goringe MJ. High voltage electron microscopy. In:Peter Roland Swann, editors. Blackwell Scientific Publications; 1973. p.365. Available from: https://web.cecs.pdx.edu/~cgshirl/Glenns%20Publications/02%201974%20Critical%20Voltage%20Effect%20and%20Applications%20Thomas%20Shirley%20Lally%20Fisher.pdf [Last accessed on 8 Oct 2024].
9. Tejada A, den Dekker AJ. A comparison between minimum variance control and other online compensation methods for specimen drift in transmission electron microscopy. Multidim Syst Sign Process 2014;25:247-71.
10. Baker R, Feates F, Harris P. Continuous electron microscopic observation of carbonaceous deposits formed on graphite and silica surfaces. Carbon 1972;10:93-6.
11. Baker R. Nucleation and growth of carbon deposits from the nickel catalyzed decomposition of acetylene. Journal of Catalysis 1972;26:51-62.
12. Baker RTK, Harris PS. Controlled atmosphere electron microscopy. J Phys E: Sci Instrum 1972;5:793-7.
13. Fujita H, Komatsu M, Ishikawa I. A universal environmental cell for a 3MV-class electron microscope and its applications to metallurgical subjects. Jpn J Appl Phys 1976;15:2221-8.
14. Hashimoto H, Naiki T, Eto T, Fujiwara K. High temperature gas reaction specimen chamber for an electron microscope. Jpn J Appl Phys 1968;7:946.
15. Hiziya K, Hashimoto H, Watanabe M, Mihama K. Gas reaction on the specimen. In: Bargmann W, Möllenstedt G, Niehrs H, Peters D, Ruska E, Wolpers C, editors. Verhandlungen physikalisch-technischer teil. Berlin: Springer Berlin, Heidelberg; 1960. pp. 80-2.
16. Hashimoto H, Kumao A, Eto T, Fujiwara K. Drops of oxides on tungsten oxide needles and nuclei of dendritic crystals. Journal of Crystal Growth 1970;7:113-6.
17. Venables JA, Ball DJ, Thomas GJ. An electron microscope liquid helium stage for use with accessories. J Sci Instrum 1968;1:121-6.
18. Bostanjoglo O, Lischke B. Elektronenmikroskopische untersuchungen am kondensierten wasserstoff, stickstoff und sauerstoff. Zeitschrift für Naturforschung A 1967;22:1620-2.
19. Honjo G, Kitamura N, Shimaoka K, Mihama K. Low temperature specimen method for electron diffraction and electron microscopy. J Phys Soc Jpn 1956;11:527-36.
20. Venables JA. Liquid helium cooled tilting stage for an electron microscope. Review of Scientific Instruments 1963;34:582-3.
21. Piercy GR, Gilbert RW, Howe LM. A liquid helium cooled finger for the Siemens electron microscope. J Sci Instrum 1963;40:487-9.
22. Goringe MJ, Valdrè U. Use of the bright field shadow technique to study superconductivity in the electron microscope. Philosophical Magazine 1963;8:1999-2003.
23. Boersch H, Bostanjoglo O, Niedrig H. Temperaturabhängigkeit der transparenz dünner schichten für schnelle elektronen. Z Physik 1964;180:407-14.
24. Boersch H, Niedrig H, Yersin H. Temperature dependence of large angle electron scattering at polycrystalline gold foils. Physics Letters A 1967;25:195-6.
25. Watanabe H, Ishikawa I. A liquid helium cooled stage for an electron microscope. Jpn J Appl Phys 1967;6:83.
26. Heide HG, Urban K. A novel specimen stage permitting high-resolution electron microscopy at low temperatures. J Phys E: Sci Instrum 1972;5:803-8.
27. Chlebek HG, Curzon AE. A liquid helium stage for the Philips EM 300 electron microscope. J Phys E: Sci Instrum 1973;6:1105-6.
28. Nogales E. The development of cryo-EM into a mainstream structural biology technique. Nat Methods 2016;13:24-7.
29. Fernández-Morán H. Low-temperature preparation techniques for electron microscopy of biological specimens based on rapid freezing with liquid helium II. Ann N Y Acad Sci 1960;85:689-713.
30. Taylor KA, Glaeser RM. Electron diffraction of frozen, hydrated protein crystals. Science 1974;186:1036-7.
31. Chiu W. Electron microscopy of frozen, hydrated biological specimens. Annu Rev Biophys Biophys Chem 1986;15:237-57.
32. Dubochet J, Mcdowall A. Vitrification of pure water for electron microscopy. Journal of Microscopy 1981;124:3-4.
33. Dubochet J, Adrian M, Chang JJ, et al. Cryo-electron microscopy of vitrified specimens. Q Rev Biophys 1988;21:129-228.
34. Adrian M, Dubochet J, Lepault J, McDowall AW. Cryo-electron microscopy of viruses. Nature 1984;308:32-6.
35. Bostanjoglo O, Röhkel K. Superferromagnetism in thin Gd and Gd-Au films. Phys Stat Sol (a) 1971;7:387-92.
36. Gai PL. In-situ environmental transmission electron microscopy. In: Kirkland A, Haigh S, Kroto H, O’brien P, Craighead H, editors. Nanocharacterisation. The Royal Society of Chemistry; 2007. pp. 268-290.
37. Gai PL, Boyes ED. Dynamic in situ experiments in a 1Å double aberration corrected environment. In: Luysberg M, Tillmann K, Weirich T, editors. EMC 2008 14th european microscopy congress 1–5 september 2008, Aachen, Germany. Berlin: Springer Berlin Heidelberg; 2008. pp. 479-80.
38. Kamino T, Saka H. A newly developed high resolution hot stage and its application to materials characterization. Microsc Microanal Microstruct 1993;4:127-35.
39. Gai PL, Boyes ED. Advances in atomic resolution in situ environmental transmission electron microscopy and 1A aberration corrected in situ electron microscopy. Microsc Res Tech 2009;72:153-64.
40. Tai K, Liu Y, Dillon SJ. In situ cryogenic transmission electron microscopy for characterizing the evolution of solidifying water ice in colloidal systems. Microsc Microanal 2014;20:330-7.
42. Hotz MT, Corbin G, Dellby N, et al. Optimizing the Nion STEM for in-situ experiments. Microsc Microanal 2018;24:1132-3.
43. Goodge BH, Bianco E, Schnitzer N, Zandbergen HW, Kourkoutis LF. Atomic-resolution cryo-STEM across continuously variable temperatures. Microsc Microanal 2020;26:439-46.
44. Fernandez-Leiro R, Scheres SH. Unravelling biological macromolecules with cryo-electron microscopy. Nature 2016;537:339-46.
45. Ross FM. Opportunities and challenges in liquid cell electron microscopy. Science 2015;350:aaa9886.
46. Dubochet J. On the development of electron cryo-microscopy (nobel lecture). Angew Chem Int Ed Engl 2018;57:10842-6.
47. Cui Y, Kourkoutis L. Imaging sensitive materials, interfaces, and quantum materials with cryogenic electron microscopy. Acc Chem Res 2021;54:3619-20.
48. Zachman MJ, Tu Z, Choudhury S, Archer LA, Kourkoutis LF. Cryo-STEM mapping of solid-liquid interfaces and dendrites in lithium-metal batteries. Nature 2018;560:345-9.
49. Fan Z, Zhang L, Baumann D, et al. In situ transmission electron microscopy for energy materials and devices. Adv Mater 2019;31:e1900608.
50. Liang J, Xiao X, Chou TM, Libera M. Analytical cryo-scanning electron microscopy of hydrated polymers and microgels. Acc Chem Res 2021;54:2386-96.
51. Gong X, Gnanasekaran K, Chen Z, et al. Insights into the structure and dynamics of metal-organic frameworks via transmission electron microscopy. J Am Chem Soc 2020;142:17224-35.
52. Li Y, Zhou W, Li Y, et al. Unravelling atomic structure and degradation mechanisms of organic-inorganic halide perovskites by cryo-EM. Joule 2019;3:2854-66.
53. Hart JL, Cha JJ. Seeing quantum materials with cryogenic transmission electron microscopy. Nano Lett 2021;21:5449-52.
54. El Baggari I, Savitzky BH, Admasu AS, et al. Nature and evolution of incommensurate charge order in manganites visualized with cryogenic scanning transmission electron microscopy. Proc Natl Acad Sci U S A 2018;115:1445-50.
55. Bianco E, Kourkoutis LF. Atomic-resolution cryogenic scanning transmission electron microscopy for quantum materials. Acc Chem Res 2021;54:3277-87.
56. Jiang T, Ni F, Recalde-benitez O, et al. Observation of dislocation-controlled domain nucleation and domain-wall pinning in single-crystal BaTiO3. Applied Physics Letters 2023;123:202901.
57. Ignatans R, Damjanovic D, Tileli V. Local hard and soft pinning of 180° domain walls in BaTiO3 probed by in situ transmission electron microscopy. Phys Rev Materials 2020;4:104403.
58. Ignatans R, Damjanovic D, Tileli V. Individual barkhausen pulses of ferroelastic nanodomains. Phys Rev Lett 2021;127:167601.
59. O’Reilly T, Holsgrove K, Arredondo M. Investigating BaTiO3 via in situ heating TEM. Microsc. Electron Ion Microsc 2021. Available from: https://analyticalscience.wiley.com/content/article-do/investigating-batio3-via-situ-heating-tem [Last accessed on 21 Sep 2024].
60. O’Reilly T, Holsgrove KM, Zhang X, et al. The effect of chemical environment and temperature on the domain structure of free-standing BaTiO3 via in situ STEM. Adv Sci (Weinh) 2023;10:e2303028.
61. Tsuda K, Sano R, Tanaka M. Nanoscale local structures of rhombohedral symmetry in the orthorhombic and tetragonal phases of BaTiO3 studied by convergent-beam electron diffraction. Phys Rev B 2012;86:214106.
62. Mun J, Peng W, Roh CJ, et al. In situ cryogenic HAADF-STEM observation of spontaneous transition of ferroelectric polarization domain structures at low temperatures. Nano Lett 2021;21:8679-86.
63. Tyukalova E, Vimal Vas J, Ignatans R, et al. Challenges and applications to operando and in situ TEM imaging and spectroscopic capabilities in a cryogenic temperature range. Acc Chem Res ;2021:3125-35.
64. Wang YL, He ZB, Damjanovic D, Tagantsev AK, Deng GC, Setter N. Unusual dielectric behavior and domain structure in rhombohedral phase of BaTiO3 single crystals. Journal of Applied Physics 2011;110:014101.
65. Recalde-Benitez O, Pivak Y, Jiang T, et al. Weld-free mounting of lamellae for electrical biasing operando TEM. Ultramicroscopy 2024;260:113939.
66. Pivak Y, Sun H, van Omme T, et al. Development of a stable cryogenic in situ biasing system for atomic resolution (s)TEM. Microsc Microanal 2023;29:1695.
67. Omme JT, Zakhozheva M, Spruit RG, Sholkina M, Pérez Garza HH. Advanced microheater for in situ transmission electron microscopy; enabling unexplored analytical studies and extreme spatial stability. Ultramicroscopy 2018;192:14-20.
68. Krisper R, Lammer J, Pivak Y, Fisslthaler E, Grogger W. The performance of EDXS at elevated sample temperatures using a MEMS-based in situ TEM heating system. Ultramicroscopy 2022;234:113461.
69. Yang Y, Vijayan S, Yesibolati MN, Jinschek JR. Standard calibrations and prediction for thermal gradients during in situ transmission electron microscopy heating experiments. Microscopy and Microanalysis 2024;30:ozae044.824.
70. Vijayan S, Wang R, Kong Z, Jinschek JR. Quantification of extreme thermal gradients during in situ transmission electron microscope heating experiments. Microsc Res Tech 2022;85:1527-37.
71. Molina-Luna L, Wang S, Pivak Y, et al. Enabling nanoscale flexoelectricity at extreme temperature by tuning cation diffusion. Nat Commun 2018;9:4445.
72. Merz WJ. The electric and optical behavior of BaTiO3 single-domain crystals. Phys Rev 1949;76:1221.
73. Zhuo F, Zhou X, Gao S, et al. Anisotropic dislocation-domain wall interactions in ferroelectrics. Nat Commun 2022;13:6676.
74. Fujii I, Trolier-mckinstry S. Temperature dependence of dielectric nonlinearity of BaTiO3 ceramics. Microstructures 2023:3.
75. Hershkovitz A, Johann F, Barzilay M, Hendler Avidor A, Ivry Y. Mesoscopic origin of ferroelectric-ferroelectric transition in BaTiO3: orthorhombic-to-tetragonal domain evolution. Acta Materialia 2020;187:186-90.
76. Zhuo F, Zhou X, Gao S, et al. Intrinsic-strain engineering by dislocation imprint in bulk ferroelectrics. Phys Rev Lett 2023;131:016801.
77. Wu H, Zhu J, Zhang T. Pseudo-first-order phase transition for ultrahigh positive/negative electrocaloric effects in perovskite ferroelectrics. Nano Energy 2015;16:419-27.
78. Li YL, Hu SY, Choudhury S, et al. Influence of interfacial dislocations on hysteresis loops of ferroelectric films. Journal of Applied Physics 2008;104:104110.
79. Zhang S, Li F, Jiang X, Kim J, Luo J, Geng X. Advantages and challenges of relaxor-PbTiO3 ferroelectric crystals for electroacoustic transducers - a review. Prog Mater Sci 2015;68:1-66.
80. Marton P, Rychetsky I, Hlinka J. Domain walls of ferroelectric BaTiO3 within the ginzburg-landau-devonshire phenomenological model. Phys Rev B 2010;81:144125.
81. Chou J, Lin M, Lu H. Ferroelectric domains in pressureless-sintered barium titanate. Acta Materialia 2000;48:3569-79.
82. Williams DB, Carter CB. Transmission electron microscopy: a textbook for materials science. 2nd ed. Springer; 2008.
83. Höfling M, Zhou X, Riemer LM, et al. Control of polarization in bulk ferroelectrics by mechanical dislocation imprint. Science 2021;372:961-4.
84. Everhardt AS, Damerio S, Zorn JA, et al. Periodicity-doubling cascades: direct observation in ferroelastic materials. Phys Rev Lett 2019;123:087603.
85. Acosta M, Novak N, Rojas V, et al. BaTiO3-based piezoelectrics: fundamentals, current status, and perspectives. Applied Physics Reviews 2017;4:041305.
86. Qiu C, Wang B, Zhang N, et al. Transparent ferroelectric crystals with ultrahigh piezoelectricity. Nature 2020;577:350-4.
87. Nataf GF, Guennou M, Gregg JM, et al. Domain-wall engineering and topological defects in ferroelectric and ferroelastic materials. Nat Rev Phys 2020;2:634-48.
88. Hlinka J, Márton P. Phenomenological model of a 90° domain wall in BaTiO3-type ferroelectrics. Phys Rev B 2006;74:104104.
89. Erhart J, Cao W. Permissible symmetries of multi-domain configurations in perovskite ferroelectric crystals. Journal of Applied Physics 2003;94:3436-45.
90. Gao J, Xue D, Liu W, Zhou C, Ren X. Recent progress on BaTiO3-based piezoelectric ceramics for actuator applications. Actuators 2017;6:24.
91. Ren X. Large electric-field-induced strain in ferroelectric crystals by point-defect-mediated reversible domain switching. Nat Mater 2004;3:91-4.
92. Zhuo F, Zhou X, Dietrich F, et al. Dislocation density-mediated functionality in single-crystal BaTiO3. Adv Sci (Weinh) 2024;11:e2403550.
Cite This Article
How to Cite
Jiang, T.; Pivak Y.; Ni F.; van der Gugten G.; Li J.; Zhuo F.; Molina-Luna L. Micro-electromechanical system-based cryogenic and heating in situ transmission electron microscopy for investigating phase transitions and domain evolution in single-crystal BaTiO3. Microstructures. 2024, 4, 2024058. http://dx.doi.org/10.20517/microstructures.2024.50
Download Citation
Export Citation File:
Type of Import
Tips on Downloading Citation
Citation Manager File Format
Type of Import
Direct Import: When the Direct Import option is selected (the default state), a dialogue box will give you the option to Save or Open the downloaded citation data. Choosing Open will either launch your citation manager or give you a choice of applications with which to use the metadata. The Save option saves the file locally for later use.
Indirect Import: When the Indirect Import option is selected, the metadata is displayed and may be copied and pasted as needed.
About This Article
Copyright
Data & Comments
Data
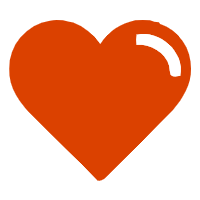
Comments
Comments must be written in English. Spam, offensive content, impersonation, and private information will not be permitted. If any comment is reported and identified as inappropriate content by OAE staff, the comment will be removed without notice. If you have any queries or need any help, please contact us at support@oaepublish.com.