Cancer-associated fibroblast-derived extracellular vesicles: regulators and therapeutic targets in the tumor microenvironment
Abstract
Cancer-associated fibroblasts (CAFs) constitute a critical component of the tumor microenvironment (TME). CAFs can be reprogrammed by cancer cells, leading to the production of extracellular vesicles (EVs). These EVs serve as carriers for bioactive substances, including proteins, nucleic acids, and metabolic products, thereby facilitating tumor progression. CAF-derived EVs exert substantial influence on tumor cell proliferation, invasion, and metastasis, the immunological environment, and the processes of lymphangiogenesis and angiogenesis. Despite their potential as non-invasive biomarkers and therapeutic delivery vehicles, the clinical application of CAF-derived EVs is currently limited by challenges in purification and precise targeting. This review delineates the diverse roles of CAF-derived EVs in tumor growth, metastasis, and immune evasion within the TME.
Keywords
INTRODUCTION
The tumor microenvironment (TME) refers to the internal milieu within which tumor cells proliferate and subsist, which consists of various cellular components and non-cellular components. Cellular components consist of immune cells and stromal cells. Among the immune cells are T and B lymphocytes, tumor-associated macrophages (TAMs), dendritic cells, natural killer cells, neutrophils, and myeloid-derived suppressor cells (MDSCs), and so forth. Stromal cells comprise tumor-associated fibroblasts [cancer-associated fibroblasts (CAFs)], pericytes, endotheliocytes, mesenchymal stromal cells, and the like; non-cellular components encompass extracellular matrix (ECM) and other secreted molecules such as growth factors, cytokines, chemokines, and extracellular vesicles (EVs), as well as the blood and lymphatic vascular network[1]. The composition of the TME is quite complex and is a dynamic and variable process. It was once considered bystanders of tumorigenesis but is now known to play critical roles in the pathogenesis of cancer. However, mechanistic studies, including those involving preclinical tumor models, have shown that cancer development and progression occur alongside alterations in the surrounding stroma. Cancer cells and all other cells in the TME can functionally reprogram their microenvironment by secreting various cytokines, chemokines, and other factors, enabling them to play a determinative role in tumor survival and progression. The cellular composition and functional state of the TME can differ extensively depending on the organ in which the tumor arises, the intrinsic features of cancer cells, the tumor stage, and patient characteristics[1-3]. In recent years, researchers have been exploring a new cancer treatment modality: targeting the tumor stroma.
CAFs are fibroblasts found within the TME near cancer cells and consist of multiple subtypes with distinct functions, demonstrating significant plasticity. Research suggests that CAFs are derived from different origins, such as pre-existing quiescent stellate cells and normal fibroblasts (NFs), bone marrow-derived fibroblasts and mesenchymal stem cells (MSCs), endothelial cells, epithelial cells, along with pericytes, smooth muscle cells, and adipocytes[4]. Based on the expression of certain markers, classifications of CAFs broadly converge on three main subtypes: myofibroblastic CAFs (myCAFs), inflammatory CAFs (iCAFs), and antigen-presenting CAFs (apCAFs). These subtypes undergo alterations during tumor progression and are regulated at the spatial level. For instance, in pancreatic cancer, three distinct CAF subtypes coexist and possess different functional characteristics and transcriptomic plasticity. The functions of myCAFs and iCAFs rely on the secretion of ECM and immunomodulatory factors, respectively, while apCAFs interact directly with T cells to promote T cell exhaustion[2,5]. CAFs are activated by inflammatory mediators within the TME, encompassing soluble factors generated by the tumor, such as transforming growth factor beta (TGF-β), interleukin-1 (IL-1), interleukin-6 (IL-6), and tumor necrosis factor-alpha (TNF-α)[6,7]. Furthermore, cancer cells can transform dormant NFs into CAFs through direct cell-to-cell communication. For example, cancerous pre-mammary ductal cells can also activate CAFs in situ through direct epithelial-stromal interactions mediated by Jagged1/Notch2 ligand-receptor binding[8]. Meanwhile, CAFs synthesize and remodel the ECM, modify the mechanical properties of the ECM, and alter the behaviors of cancer cells and immune cells. Additionally, CAFs have an impact on angiogenesis, possess robust immunomodulatory capabilities, and contribute to the immune evasion of cancer cells. CAFs interact extensively with cancer cells and can influence other components of the TME, such as the ECM and the immune infiltrates. Histopathological analysis has shown that the abundance of CAFs is related to prognosis among different human cancers[6,7,9,10]. CAFs also modulate the efficacy of therapies and constitute a therapy target in their own right.
EVs are particles released from cells that are enclosed by a lipid bilayer and do not contain functional nuclei. EVs can be derived from a variety of eukaryotic cells, such as tumor cells, immune cells, and stem cells, as well as prokaryotic cells, including Gram-positive and Gram-negative bacteria. Moreover, EVs can be isolated from multiple body fluids and solid tissues, including blood, urine, cerebrospinal fluid, saliva, synovial fluid, and brain or tumor tissues[11,12]. EVs have a broad range of biological functions and participate in multiple physiological and pathological processes. Their ability to mediate intercellular communication by transferring a wide spectrum of molecules between cells gives them an important role in complex biological processes such as tumorigenesis, inflammation, immune response modulation, tissue repair, and apoptosis[13-15]. In recent years, there has been growing evidence that CAFs are an important source of EVs in the TME, and CAF-derived EVs have been recognized as crucial mediators in regulating the extracellular communication between CAFs and cancer cells, and can affect tumor progression in multiple ways[16,17].
OVERVIEW OF EVs
According to the Minimal information for studies of extracellular vesicles 2023 (MISEV2023) guidelines, EVs can be divided into different subtypes based on their size, density, molecular composition, or cell origin, among other characteristics. For example, EVs can be classified into small EVs and large EVs based on the diameter of the separated particles. Small EVs are often described as < 200 nm in diameter. Additionally, EVs can be classified into exosomes and ectosomes based on their biogenesis pathways. Exosomes are EVs from internal compartments of the cell that are released via the multivesicular bodies (MVBs), while ectosomes are EVs from the cell surface. Additionally, ectosomes are enriched for CD9 and CD81, while exosomes are enriched in CD63, CD9, CD81, Alix, and syntenin[11,18,19]. Owing to the difficulty in isolating pure exosomes from cells in most instances and the ambiguity regarding the source of the isolated EVs, we term the exosomes mentioned in research articles “small Evs” or “Evs” rather than “exosomes”.
Synthesis and release of EVs
Exosomes originate from the endosomal pathway by the formation of the early sorting endosomes (ESEs), late sorting endosomes (LSEs), and ultimately MVBs, which contain intraluminal vesicles (ILVs). Finally, MVBs fuse with the plasma membrane, and exosomes are released. Through endocytosis and plasma membrane invagination, fluid and extracellular constituents such as proteins, lipids, metabolites, small molecules, and ions can enter cells, along with cell surface proteins. However, ectosomes are vesicles that pinch off the surface of the plasma membrane via outward budding, and include microvesicles, microparticles, and large EVs in the size range of 50 to 1 mm in diameter. During EV synthesis, donor cells’ cytoplasm is loaded into EVs and secreted into the extracellular environment[20-23] [Figure 1]. After successfully released into the extracellular environment, EVs can be taken up by interacting with receptors and ligands, thereby mediating material transport and information transmission between the TME and tumor cells[24-26].
Figure 1. Biogenesis of extracellular vesicles and the structure and contents of exosomes. The formation of exosomes included four steps: (i) ESEs formation: Through the mechanisms of endocytosis and invagination of the plasma membrane, ESEs are formed, a process energized by Mt; (ii) LSE formation: After cytoplasmic sorting, ESEs mature and eventually form LSEs. During the formation of ESEs and LSEs, they are capable of exchanging materials with the cell nucleus, ER, and GA; (iii) MVB formation: LSEs’ membrane buds inwardly to form multiple ILVs (future exosomes), which eventually transform into MVBs; (iv) Release: MVBs fuse with the plasma membrane, and ILVs released to extracellular space are called exosomes. Ectosomes are vesicles that pinch off the surface of the plasma membrane via outward budding. Exosomes have a diameter of 30-150 nm and a bilayer membrane structure, while ectosomes range from 50 to 1 mm in diameter. EVs are highly heterogeneous bilayer membrane structures carrying diverse cargos, such as proteins, nucleic acids, and lipids, and their content can vary significantly across different cells and conditions. ESEs: Early sorting endosomes; Mt: mitochondria; LSEs: late sorting endosomes; MVBs: multivesicular bodies; ILVs: intraluminal vesicles; ER: endoplasmic reticulum; GA: Golgi apparatus; SHH: Sonic Hedgehog; Evs: extracellular vesicles; TGF-β: transforming growth factor beta; IL-6: interleukin-6; TCA: tricarboxylic acid.
Contents and functions of EVs
EVs are highly heterogeneous, which is reflected in differences in their contents. EVs can carry various cargoes, including proteins, nucleic acids, and lipids, and this content can vary widely between cells and conditions. In addition to carrying bioactive contents, EVs are also involved in tumor cell proliferation, metastasis, drug resistance, and immune response[27-29] [Figure 1].
Proteins are important components of EVs contents. There are multiple protein families in EVs, such as receptors, transcription factors, enzymes, and ECM proteins[30]. EVs from different sources have different characteristics[31]. Proteins in EVs from CAFs can participate in tumorigenesis by activating signaling pathways in recipient cells. For example, Sonic Hedgehog (SHH) in CAF-derived EVs can promote the proliferation and migration of esophageal squamous cell carcinoma (ESCC)through Hedgehog signaling[32]. Additionally, Valbona Luga et al. found that tumor cells endocytose CD81-containing EVs from CAFs, which could activate the Wnt/β-catenin pathway to promote metastasis[33].
Accumulating evidence has recently shown that exosome-derived ncRNAs are vital for tumor progression[34]. ncRNAs mainly include miRNAs, lncRNAs, and circRNAs. They mainly bind to the 3’ untranslated region of target mRNA, inhibiting the translation and expression of target genes, thereby affecting the incidence and development of tumors[35,36].
Metabolites derived from EVs affect the behavior of malignant tumors, and they can be divided into three major categories (1) amino acids: glutamine, threonine, serine, and valine; (2) lipids: palmitate and stearate; (3) tricarboxylic acid cycle intermediates: citrate, pyruvate, α-ketoglutarate, fumarate, and malate[37]. Tumor metabolic reprogramming is one of the important features of cancer[38], with the upregulation of glycolysis, glutaminolysis, lipid metabolism, mitochondrial biogenesis, pentose phosphate pathway, and other biosynthetic and bioenergetic pathways[39], which leads to the rapid proliferation, survival, invasion, metastasis, and resistance to treatment of tumor cells[40].
EVs from NFs and CAFs
In physiological conditions, fibroblasts are the quintessential supporting cell type and usually quiescent as shown by their low levels of cell proliferation and metabolic activity. They are present in all tissues, where they adapt to unique microenvironmental cues and cater to the needs of the specialized surrounding cells to help control tissue homeostasis and organ function. NFs in normal tissues produce ECM, which is vital to maintaining structural support and positional information for neighboring cells and offering a medium for cytokines, growth factors, and metabolites[5,9,10]. During tissue injury or inflammatory response, fibroblasts can be activated, with enhanced cell proliferation and metabolic activity, including protein synthesis. Activated fibroblasts are one of the key effector cell types in the wound healing process, which presumably promote wound healing via secreted factors including EVs[41,42]. Oh et al. successfully isolated and purified L929-EV from fibroblasts. It can accelerate wound healing in the mouse model of skin trauma by promoting fibroblast proliferation and migration, along with endothelial cell migration and lumen formation[43].
In certain conditions, the NFs can establish self-activating and feedback loops and transform into CAFs, which is crucial for the survival, proliferation, and invasion of cancer cells. For example, EVs secreted by CAFs differ in characteristics and biological functions from those secreted by NFs[10]. Specifically, EVs from CAFs typically carry large amounts of inhibitory cytokines (e.g., TGF-β) and death receptor ligands (e.g., PD-L1)[45]. Hu et al.’s study found that, compared with the EVs secreted by NFs, those secreted by CAFs increased expression of miR-92a-3p activates the Wnt/β-catenin pathway and inhibits mitochondrial apoptosis by directly inhibiting FBXW7 and MOAP1, contributing to cell stemness, EMT, metastasis, and 5-FU/L-OHP resistance in colorectal cancer (CRC)[46]. Additionally, Wang et al. found that in head and neck squamous cell carcinoma (HNSCC), CAF-derived EVs contain lower levels of miRNA-3188 compared to those derived from NFs[47]. In the TME, CAF-derived EVs also regulate tumor growth, metastasis, and angiogenesis, and mediate therapy resistance of tumor cells.
REGULATORY MECHANISMS OF CAF-DERIVED EVS
CAF-derived EVs regulate tumor proliferation
The capacity for unlimited cell division is a cardinal feature distinguishing malignant tumor cell populations from their benign counterparts[38]. Accumulating evidence suggests that ncRNAs, particularly miRNAs, regulate tumor cell proliferation through modulation of signaling pathways[48] [Figure 2A]. For instance, miRNA-20a has been found to inhibit the PTEN/PI3K-AKT pathway in non-small cell lung cancer (NSCLC), which promotes the proliferation of tumor cells[49]. Transfer of miRNA-500a-5p from CAFs to cancer cells in breast cancer (BC) stimulates proliferation and metastasis through binding to ubiquitin-specific peptidase 28 (USP28)[50]. Furthermore, it has been reported that under nutrient deprivation or nutrient stress conditions, CAF-derived EVs might inhibit mitochondrial oxidative phosphorylation and enhance glutamine-dependent reductive carboxylation and glycolysis, which promotes tumor growth[40].
Figure 2. Contents and functions of CAF-derived EVs. (A) CAF-derived EVs regulate tumor proliferation; (B) CAF-derived EVs regulate tumor invasion and metastasis; (C) CAF-derived EVs regulate the tumor immune microenvironment; (D) CAF-derived EVs regulate tumor angiogenesis/lymphangiogenesis. CAF: Cancer-associated fibroblasts; EVs: extracellular vesicles; DC: dendritic cell; Mac: macrophage; Fb: fibroblast; Endo: endotheliocyte; TME: tumor microenvironment; IL-6: interleukin-6; CDEs: CAF-derived EVs.
In addition to promoting the proliferation, certain substances in EVs can also inhibit the proliferation. A study by Xu et al. discovered that miRNA-139 in gastric cancer (GC) can inhibit development and metastasis by reducing the levels of matrix metalloproteinase 11 (MMP11) in the TME[51]. Wang et al. found that CAF-derived EVs in HNSCC contain lower levels of miRNA-3188 compared to those derived from NFs[47]. Similarly, in hepatocellular carcinoma (HCC), miRNA-150-3p[52] and miRNA-320a[53] in EVs have been found to be significantly reduced. The reduction in or absence of these miRNAs has been shown to promote tumor proliferation, invasion, and metastasis. Therefore, the potential therapeutic value of CAF-derived EVs in inhibiting tumor growth warrants further investigation.
CAF-derived EVs regulate tumor invasion and metastasis
Metastasis is a significant cause of poor prognosis and mortality in cancer patients. It involves the dissemination of tumor cells to distant tissues and subsequent adaptation and survival in new microenvironments. Generally, the process of metastasis consists of four stages: local invasion and intravasation, survival in circulation, extravasation, and ultimately colonization at a new location[54,55]. Among the most critical strategies for metastasis is the promotion of tumor dissemination through EV-ncRNAs [Figure 2B].
In OSCC, the miR-34a-5p/AXL axis promotes β-catenin’s nuclear translocation and induces the EMT, which in turn leads to SNAIL’s transcriptional upregulation and the activation of MMP-2 and MMP-9[56]. The upregulation of MMP expression accelerates cancer invasion and metastasis, as well as poor prognoses[57]. Wu et al. found that the knockdown of the focal adhesion kinase (FAK) gene in CAFs promoted the migration of tumor cells. Analysis of miRNAs within CAF-derived EVs revealed multiple changes in EV-ncRNAs in FAK-deficient CAFs, such as the miRNA-16 and miRNA-148a. It is the upregulation of miRNA-16 and miRNA-148a that promotes the invasion and metastasis[58]. However, the underlying mechanisms remain unclear.
Additionally, research indicates that in the early stages of cancer, CAF-derived EVs may facilitate the establishment of a pre-metastatic microenvironment (PMN), thereby enhancing the likelihood of metastatic tumor cells successfully surviving and colonizing in foreign microenvironments. In salivary adenoid cystic carcinoma (SACC), CAF-derived EVs with upregulation of plasma IL-6 and integrin β1 possess robust stromal remodeling capabilities, inducing the formation of PMN in the lungs of mice and increasing pulmonary metastasis of SACC. Mechanistically, IL-6 activates the JAK2/STAT3 signaling pathway, which promotes EMT in SACC, thereby forming PMN to facilitate tumor metastasis[59]. In contrast, integrin β1 promotes the uptake of CAF-derived EVs by lung fibroblasts, further facilitating the formation of pulmonary PMN[14]. These findings enhance our understanding of the mechanisms underlying tumor invasion and metastasis, offering novel insights for future research in the domain of CAF-derived EVs.
CAF-derived EVs regulate the TME
The inhibitory TME plays a pivotal role in determining the fate of tumors. During the initial stages of tumor development, the immune system can act as an antitumor defense. However, in later stages, tumor cells develop various mechanisms to evade immune surveillance. Despite recent advances in immunotherapy, the ability of tumors to evade immune surveillance has consistently been recognized as a barrier to the success of tumor immunotherapy [Figure 2C][60].
Researchers have shown that CAF-derived EVs can also contribute to the creation of an immunosuppressive microenvironment. Specifically, they can exhaust T cells in tumors and hinder their ability to perform essential immune functions. Feng et al. demonstrated that CAFs-EVs facilitate immune evasion in bladder cancer (BLCA) by upregulating the PD-L1/PD-1 pathway. Specifically, CAFs-EVs not only decrease apoptosis and enhance invasion in BLCA cells but also impair CD8+ T cell function by delivering PD-L1, thereby reducing CD8+ T cell proliferation and infiltration. Additionally, CAFs-EVs lower the secretion of key cytokines such as IFN-γ, IL-2, and TNF-α from CD8+ T cells[61]. Furthermore, through STAT3 activation, miRNA-21 in CAF-derived EVs promotes the production of MDSCs. MDSCs are a type of immunosuppressive cells capable of inhibiting the proliferation of CD8+ T cells and contributing to the establishment of an immunosuppressive microenvironment[62,63].
On the other hand, Wang et al.’s study revealed that EVs in oral squamous cell carcinoma (OSCC) not only reduce CD3+ and CD8+ immune cells in tumor tissue, but also shape an immunosuppressive microenvironment by influencing the expression of immune genes. CAF-derived EVs contain numerous substances related to immune regulation, including has-miRNA-139-5p, EIF6, and PECAM1 miRNA, which regulate the expression of target cell proteins, including PIGR, CD81, UACA, and PTTG1IP, in cancer-related pathways, playing a significant role in regulating immune responses and promoting OSCC growth[64]. Currently, there is limited research on the impact of EVs on immune cells in the TME. Nevertheless, these studies provide a fresh perspective on the role of CAF-derived EVs in tumors and comprehensively reveal the immune microenvironment regulated by CAFs. Investigating the mechanisms of action of CAF-derived EVs in the tumor immune microenvironment represents a promising avenue for predicting prognosis and developing cancer treatment strategies.
CAF-derived EVs regulate tumor angiogenesis/lymphangiogenesis
Tumors proliferate rapidly and require new blood/lymphatic vessel networks to obtain nutrients for growth, but this can also increase the risk of metastasis[65]. It has been demonstrated that CAF-derived EVs are crucial in regulating angiogenesis and lymphangiogenesis [Figure 2D].
In CRC, CAFs can downregulate the levels of BMP5 and TXNIP by upregulating the levels of miRNA -522-3p in small EVs[66]. This promotes proliferation, migration, and invasion while inhibiting CRC cell apoptosis in vitro. Similarly, Wu et al. demonstrated that downregulation of miRNA-29b-1-5p in CAF-derived EVs via the VSIG1/ZO-1 axis inhibits angiogenesis, GC cell migration and invasion, as well as the progression[67]. In the study conducted by Sun et al., it is noteworthy that miRNA-21 is highly abundant in CAF-derived EVs. Moreover, miRNA-21 has been shown to significantly upregulate the expression of fibroblast activation protein and α-smooth muscle actin in NFs, thereby inducing their transformation into CAFs. The CAF-derived EVs play a crucial role in promoting angiogenesis in multiple myeloma (MM) by delivering miRNA-21 to MM endothelial cells[68].
In addition to regulating angiogenesis, CAF-derived EVs are also closely linked to the development of lymphatic vessels. For example, ESCC primarily affects the stomach and intestines and has a high potential for early lymphatic metastasis. Compared to EVs from NFs, CAF-derived EVs show significantly lower levels of miRNA-100-5p, which promotes the proliferation, migration, invasion, and lymphangiogenesis of tumor-associated lymphatic endothelial cells (TLECs). Mechanistically, miRNA-100-5p’s inhibitory effect on lymphangiogenesis may be mediated through the IGF1R/PI3K/AKT signaling axis[69]. Based on these findings, it has been observed that CAFs have the capability to secrete specific exosomes that regulate angiogenesis and lymphangiogenesis, thus opening up new avenues for research in this field.
Conclusively, CAF-derived EVs exert a pronounced regulatory influence on tumor initiation, progression, invasion, metastasis, immune evasion, and angiogenesis. The molecules, their mechanisms of action within these processes, and their biological functions are summarized in Table 1.
The role and regulatory mechanism of CAF-derived EVs
Cancer type | Molecule | Expression | Mechanism | Function | Ref. |
BC | miRNA-6, miRNA-148a | Upregulated | Through the FAK signaling pathway | Promotes invasion and metastasis | [58] |
BC | miRNA-500 a-5p | Upregulated | Binds to USP28 | Promotes proliferation and metastasis | [50] |
BC | mtDNA | Upregulated | Restores oxidative phosphorylation of cancer cells, and activates cancer stem-like cells | Endocrine therapy resistance | [40] |
CRC | miRNA-522-3p, BMP5, TXNIP | Upregulated | Inhibits the apoptosis, promotes angiogenesis | Promotes proliferation, migration, and invasion | [66] |
ESCC | miRNA-100-5p | Downregulated | Inhibits lymphangiogenesis through IGF1R/PI3K/AKT axis | Inhibit lymphatic metastasis | [69] |
ESCC | miR-21 | Upregulated | Activates STAT3 signal transduction, induces MDSCs, inhibits CD8+ T cell proliferation | Establishes the immunosuppressive TME | [62,63] |
ESCC | SHH | Upregulated | Activates the SHH signaling pathway | Promotes proliferation | [32] |
GC | miRNA-139 | Downregulated | Decreases MMP11 in the TME | Inhibits the metastasis | [51] |
GC | miRNA-29b-1-5p | Upregulated | Promotes angiogenesis through the VSIG1/ZO-1 axis | Promotes proliferation, migration, and invasion | [67] |
HCC | miRNA-150-3p, miRNA-320 a | Downregulated | Suppresses the MAPK pathway, inhibits EMT, decreases CDK2 and MMP2 | Inhibits proliferation, migration, and invasion | [52,53] |
HNSCC | miRNA-3188 | Downregulated | Targets BCL2 | Inhibits proliferation and promotes apoptosis | [47] |
LC | Integrin α2β1 | Upregulated | Promotes lung fibroblast activation and the formation of lung PMN | Creates a pre-metastatic niche, promotes the metastasis | [14] |
LC | miRNA-20 a | Upregulated | Inhibits the PTEN/PI3K/AKT pathway | Promotes the proliferation | [49] |
MM | miRNA-21 | Upregulated | Transforms NFs into CAFs, promotes tumor neovascularization | Promotes invasion | [68] |
OSCC | has-miRNA-139-5p, EIF6, PECAM1 mRNA | Upregulated | Affects the expression of immune genes, reduces CD3 and CD8+ immune cells | Establishes the immunosuppressive TME and promotes the proliferation | [64] |
OSCC | miRNA-34a-5p | Upregulated | Activates the AKT/GSK-3β/β-catenin signaling pathway, induces EMT | Promotes progression and metastasis | [56,57] |
SACC | Plasma integrin b1, IL-6 | Upregulated | Activates the JAK2/STAT3 signaling pathway, induces EMT | Promotes invasion | [59] |
POTENTIAL CLINICAL APPLICATIONS OF CAF-DERIVED EVS
Innovative tumor biomarkers
EVs hold significant potential for liquid biopsy, as the cargo of EVs derived from tumor cells not only reflects the physiological and pathological characteristics of the tumor, but also remains stable in the body fluid circulation even within the harsh TME. Consequently, proteins and nucleic acids encapsulated in exosomes can serve as early diagnostic markers for cancer. For instance, miRNA-10b enclosed within exosomes is a well-established indicator of pancreatic adenocarcinoma (PAAD) progression and is widely used for early diagnosis. Furthermore, Glypican-1 (GPC1) is specifically enriched in exosomes, and circulating exosomes with high GPC1 expression can be highly specific and sensitive when detected in the serum of PAAD patients, distinguishing between early-stage and late-stage patients with PAAD as well as healthy individuals with pancreatic diseases[70]. For example, Tian et al. analyzed the cancer-related proteome of plasma EVs from BC patients and found that the EV features were highly accurate in differentiating between metastatic and non-metastatic BC. It can precisely monitor the response to treatment and serve as an independent prognostic factor in metastatic breast cancer (MBC) patients[71]. The development of biomarkers based on EVs is intrinsically linked to the advancement of EV detection technology. When contrasted with traditional EV analysis methods, such as polymerase chain reaction (PCR), western blot (WB), EV detection methods based on fluorescence, surface-enhanced Raman spectroscopy (SERS), surface plasmon resonance (SPR), electrochemistry, and aptamers enhance the sensitivity, specificity, and practicality of EVs in clinical settings[72,73]. In conclusion, as technology progresses and research intensifies, EVs will exhibit an even more significant potential in cancer.
Drug delivery system in cancer therapy
In the field of cancer treatment, EVs are capable of enhancing the targeting capacity of cells or organs through genetic engineering and chemical modification and transferring various cargos to the target cells. The EV-mediated drug delivery technology is categorized into two types: exogenous and endogenous. Exogenous drug delivery technology refers to the isolation and purification of exosomes from cell culture supernatants or other biological fluids and the loading of the desired drugs onto the surface or within exosomes by physical or chemical means[74]. For instance, Santos et al. loaded exogenous miRNA-195-5p onto EVs. These modified EVs were absorbed by tumor cells and could reduce cell proliferation, increase cell death, and enhance targeted therapy responses in melanoma patients[74,75]. Exogenous loading is applicable when the ideal carrier is a small molecule drug or other molecules that cells cannot produce. The modification methods for EVs include passive incubation with cargo or active loading through electroporation, sonication, or freeze-thaw cycles. Meanwhile, endogenous drug delivery technology encompasses modifying donor cells via biotechnology to yield exosomes with specific products. This approach enables more precise control of the carrier content and has been employed to load therapeutic proteins, RNA, and CRISPR/Cas9 complexes into EVs[74]. For example, Katakowski et al. utilized a miRNA-146b plasmid to transfect MSCs, resulting in exosomes carrying it, which were then administered into the glioblastoma sites in mice. Their findings indicated that exosome-based therapy utilizing miRNA transport could effectively impede tumor cell proliferation[76]. Furthermore, numerous methods have been devised for the loading of siRNA into EVs, such as calcium chloride-mediated transfection, electroporation, sonication, and the employment of hydrophobically modified siRNA. For instance, in the study by Zhang et al., L9-29 mouse fibroblasts were transfected with TGF-β1 siRNA through a transfection reagent, and exosomes with TGF-β1 siRNA were isolated from the cell culture supernatant. The findings showed that compared with the use of free TGF-β1 siRNA, EVs carrying TGF-β1 siRNA effectively reduced the level of TGF-β1 in the targeted tumor cells, significantly lowering the viability and migration ability of mouse sarcoma cells[77]. The engineered CAF-derived EVs investigated by Xue et al. exhibit robust intercellular communication, transport, penetration, and targeting properties, making them a highly efficient delivery system capable of effectively overcoming the complexity and heterogeneity of the TME[78]. Similarly, there is significant potential in blocking tumor progression by regulating the secretion or level of CAF-derived EVs. For instance, the study by Sun et al. demonstrated that the application of anti-miRNA-21 or siRNA technology to lower the miRNA-21 level in CAF-derived EVs could diminish the proliferative, invasive, and metastatic capabilities of tumor cells in vitro[68]. Although the research targeting CAF-derived EVs for tumor treatment is still primarily at the animal experiment stage, it remains a potential target for cancer therapy.
Advantages and disadvantages of EVs in clinical application
EVs have shown advantages and potential in clinical applications. Firstly, EVs have a natural targeting ability, as different cell-derived EVs interact with receptor carriers through surface adhesion proteins and selectively target receptor cells through their membrane components. For example, integrin α6β4 and α6β1 can target EVs to the lungs, while integrin α6β5 can promote EV targeting to the liver[79,80]. Secondly, EVs can be derived from the patient’s own cells, such as MSCs, thereby reducing their risk of immune rejection. Moreover, EVs are incapable of self-replication, which might render them safer than stem cell transplantation in regenerative medicine[81,82]. EVs possess distinctive advantages as biomarkers for therapeutic responses or drug delivery systems. EVs encompass various biological macromolecules and play a crucial role in information transmission among different cell types. They exhibit advantages such as stability, rich content, minimally invasive sampling, higher concentrations, and enhanced stability in liquid samples, as well as existence in various types of liquid samples. This renders EVs a promising new circulating biomarker with extensive application prospects[72,83]. Additionally, EVs possess the following advantages as drug delivery vehicles. Firstly, compared to cell therapy, exosomes are safer and easier to store, and they can be separated from the patient’s body fluids and modified before reintroduction, significantly reducing the likelihood of immune reactions occurring in clinical settings[84,85]. Secondly, exosomes possess the ability to enter the cell cytosol and protect drugs (such as nucleic acids) from metabolic processes during transportation, thereby extending the drug’s circulation time and improving its stability[84]. Thirdly, exosomes are nanometer-sized vesicles that carry cell surface molecules, giving them strong penetration and inherent targeting capabilities across various biological barriers[86].
However, EVs have disadvantages in clinical applications. First, the half-life of EVs in body circulation is short, which might affect their sustained efficacy. The impact of EVs on tissues depends on their clearance rate, and macrophages have a certain clearance effect on EVs[15,83,87]. Regarding production efficiency, for regenerative therapy, EV production requires cultivating a large number of MSCs; however, the output of EVs secreted by cells is too low to meet therapeutic needs[81,82]. In terms of separation, purification, and storage, EVs have certain limitations. The currently mature methods for EV separation and purification include ultracentrifugation, immunoprecipitation or affinity purification, ultrafiltration, and size exclusion; however, the purity of purified EVs is often low, affecting subsequent experiments. The optimal storage conditions for maintaining Evs’ integrity (such as temperature or pH value) are rather demanding[15,72,83]. Nonetheless, EVs continue to demonstrate significant potential for precision cancer treatment in the future.
CONCLUSION
CAF-derived EVs have a multifaceted and pivotal impact on the TME. This comprehensive review outlines the diverse functions of CAF-derived EVs in tumorigenesis, progression, metastasis, and immune evasion. It also investigates their potential applications in cancer therapy.
Firstly, CAF-derived EVs influence tumor cell proliferation, invasion, and metastasis by transporting various biologically active molecules such as proteins, nucleic acids, and metabolic products. Notably, ncRNAs such as miRNAs and lncRNAs modulate the expression of target genes, enhancing tumor cells’ stem-like properties and EMT while improving chemoresistance and metastatic potential. Additionally, metabolic products in CAF-derived EVs contribute to reprogramming tumor metabolism by providing energy and precursor molecules for tumor growth and invasion. Secondly, CAF-derived EVs play a critical role in regulating the TIME. They can impact T cell function, facilitate MDSCs generation, and regulate the expression of immune-related genes to establish an immunosuppressive microenvironment that aids tumors in evading immune surveillance. Furthermore, the role of CAF-derived EVs in tumor angiogenesis and lymphangiogenesis warrants significant attention. By delivering specific miRNAs, CAF-derived EVs can modulate the expression of factors associated with angiogenesis, thereby impacting the vascular supply and metastatic potential of tumors.
In terms of clinical application, circulating CAF-derived EVs have shown promise as novel biomarkers, owing to their stability in bodily fluids and ability to reflect tumor characteristics through specific proteins and nucleic acids. Additionally, CAF-derived EVs can function as delivery vehicles to effectively transport therapeutic molecules to tumor cells. However, improving the targeting and purity of CAF-derived EVs remains a significant challenge in realizing their clinical utility. Lastly, it is possible to inhibit tumor proliferation and metastasis by modulating the secretion of CAF-derived EVs or the levels of their constituents, thereby providing a new avenue for therapeutic intervention.
In summary, the role of CAF-derived EVs in tumor biology is multifaceted, with broad prospects for their application in tumor diagnosis and treatment. Future research needs to further elucidate the specific mechanisms of action of CAF-derived EVs, optimize their use as biomarkers and drug carriers, and explore new targeted therapies for more effective cancer treatments.
DECLARATIONS
Acknowledgments
The authors thank Figdraw for providing scientific researchers with exquisite materials and convenient drawing platforms. All the figures in this article are made by Figdraw (www.figdraw.com).
Authors’ contributions
Provided direction and guidance throughout the preparation of this manuscript: Xie X, Chen W, Zou Y
Wrote and edited the manuscript: Xie J, Lin X, Deng X
Reviewed and made significant revisions to the manuscript: Xie J, Lin X, Deng X, Tang H
All authors confirm that they contributed to manuscript reviews, critical revision for important intellectual content, and have read and approved the final draft for submission.
All authors agree to be accountable for the content of the work.
Availability of data and materials
Not applicable.
Financial support and sponsorship
This research was funded by the National Natural Science Foundation of China (82173366, Xie X).
Conflicts of interest
Tang H is an Associate Editor on the Editorial Board of the journal Cancer Drug Resistance. Zou Y is an Editor on the Junior Editorial Board of the journal Cancer Drug Resistance. Tang H and Zou Y were not involved in any steps of editorial processing, notably including reviewer selection, manuscript handling and decision making. The other authors declared that there are no conflicts of interest.
Ethical approval and consent to participate
Not applicable.
Consent for publication
Not applicable.
Copyright
© The Author(s) 2025.
REFERENCES
1. Bejarano L, Jordāo MJC, Joyce JA. Therapeutic targeting of the tumor microenvironment. Cancer Discov. 2021;11:933-59.
2. Visser KE, Joyce JA. The evolving tumor microenvironment: from cancer initiation to metastatic outgrowth. Cancer Cell. 2023;41:374-403.
3. Hinshaw DC, Shevde LA. The tumor microenvironment innately modulates cancer progression. Cancer Res. 2019;79:4557-66.
4. Chen X, Song E. Turning foes to friends: targeting cancer-associated fibroblasts. Nat Rev Drug Discov. 2019;18:99-115.
5. Caligiuri G, Tuveson DA. Activated fibroblasts in cancer: perspectives and challenges. Cancer Cell. 2023;41:434-49.
7. Sahai E, Astsaturov I, Cukierman E, et al. A framework for advancing our understanding of cancer-associated fibroblasts. Nat Rev Cancer. 2020;20:174-86.
8. Chen Y, Zhu S, Liu T, et al. Epithelial cells activate fibroblasts to promote esophageal cancer development. Cancer Cell. 2023;41:903-18.e8.
10. Chhabra Y, Weeraratna AT. Fibroblasts in cancer: unity in heterogeneity. Cell. 2023;186:1580-609.
11. Welsh JA, Goberdhan DCI, O'Driscoll L, et al; MISEV Consortium. Minimal information for studies of extracellular vesicles (MISEV2023): from basic to advanced approaches. J Extracell Vesicles. 2024;13:e12404.
12. Colombo M, Raposo G, Théry C. Biogenesis, secretion, and intercellular interactions of exosomes and other extracellular vesicles. Annu Rev Cell Dev Biol. 2014;30:255-89.
13. Roefs MT, Sluijter JPG, Vader P. Extracellular vesicle-associated proteins in tissue repair. Trends Cell Biol. 2020;30:990-1013.
14. Kong J, Tian H, Zhang F, et al. Extracellular vesicles of carcinoma-associated fibroblasts creates a pre-metastatic niche in the lung through activating fibroblasts. Mol Cancer. 2019;18:175.
15. Escudé Martinez de Castilla P, Tong L, Huang C, et al. Extracellular vesicles as a drug delivery system: a systematic review of preclinical studies. Adv Drug Deliv Rev. 2021;175:113801.
16. Mao X, Xu J, Wang W, et al. Crosstalk between cancer-associated fibroblasts and immune cells in the tumor microenvironment: new findings and future perspectives. Mol Cancer. 2021;20:131.
17. Li C, Teixeira AF, Zhu HJ, Ten Dijke P. Cancer associated-fibroblast-derived exosomes in cancer progression. Mol Cancer. 2021;20:154.
18. Mathieu M, Névo N, Jouve M, et al. Specificities of exosome versus small ectosome secretion revealed by live intracellular tracking of CD63 and CD9. Nat Commun. 2021;12:4389.
20. Kalluri R, LeBleu VS. The biology, function, and biomedical applications of exosomes. Science. 2020;367:eaau6977.
21. Arya SB, Collie SP, Parent CA. The ins-and-outs of exosome biogenesis, secretion, and internalization. Trends Cell Biol. 2024;34:90-108.
22. Tian X, Shen H, Li Z, Wang T, Wang S. Tumor-derived exosomes, myeloid-derived suppressor cells, and tumor microenvironment. J Hematol Oncol. 2019;12:84.
23. Niel G, D'Angelo G, Raposo G. Shedding light on the cell biology of extracellular vesicles. Nat Rev Mol Cell Biol. 2018;19:213-28.
24. Yang E, Wang X, Gong Z, Yu M, Wu H, Zhang D. Exosome-mediated metabolic reprogramming: the emerging role in tumor microenvironment remodeling and its influence on cancer progression. Signal Transduct Target Ther. 2020;5:242.
25. Becker A, Thakur BK, Weiss JM, Kim HS, Peinado H, Lyden D. Extracellular vesicles in cancer: cell-to-cell mediators of metastasis. Cancer Cell. 2016;30:836-48.
26. Jiang C, Zhang N, Hu X, Wang H. Tumor-associated exosomes promote lung cancer metastasis through multiple mechanisms. Mol Cancer. 2021;20:117.
27. Valadi H, Ekström K, Bossios A, Sjöstrand M, Lee JJ, Lötvall JO. Exosome-mediated transfer of mRNAs and microRNAs is a novel mechanism of genetic exchange between cells. Nat Cell Biol. 2007;9:654-9.
28. Chen X, Liu Y, Zhang Q, et al. Exosomal miR-590-3p derived from cancer-associated fibroblasts confers radioresistance in colorectal cancer. Mol Ther Nucleic Acids. 2021;24:113-26.
29. Han QF, Li WJ, Hu KS, et al. Exosome biogenesis: machinery, regulation, and therapeutic implications in cancer. Mol Cancer. 2022;21:207.
30. Kugeratski FG, Hodge K, Lilla S, et al. Quantitative proteomics identifies the core proteome of exosomes with syntenin-1 as the highest abundant protein and a putative universal biomarker. Nat Cell Biol. 2021;23:631-41.
31. Jena BC, Mandal M. The emerging roles of exosomes in anti-cancer drug resistance and tumor progression: an insight towards tumor-microenvironment interaction. Biochim Biophys Acta Rev Cancer. 2021;1875:188488.
32. Zhao G, Li H, Guo Q, et al. Exosomal Sonic Hedgehog derived from cancer-associated fibroblasts promotes proliferation and migration of esophageal squamous cell carcinoma. Cancer Med. 2020;9:2500-13.
33. Luga V, Zhang L, Viloria-Petit AM, et al. Exosomes mediate stromal mobilization of autocrine Wnt-PCP signaling in breast cancer cell migration. Cell. 2012;151:1542-56.
34. Vokurka M, Lacina L, Brábek J, Kolář M, Ng YZ, Smetana K Jr. Cancer-associated fibroblasts influence the biological properties of malignant tumours via paracrine secretion and exosome production. Int J Mol Sci. 2022;23:964.
35. Higgs PG, Lehman N. The RNA world: molecular cooperation at the origins of life. Nat Rev Genet. 2015;16:7-17.
36. Wang J, Zhu S, Meng N, He Y, Lu R, Yan GR. ncRNA-encoded peptides or proteins and cancer. Mol Ther. 2019;27:1718-25.
37. Achreja A, Zhao H, Yang L, Yun TH, Marini J, Nagrath D. Exo-MFA - A 13C metabolic flux analysis framework to dissect tumor microenvironment-secreted exosome contributions towards cancer cell metabolism. Metab Eng. 2017;43:156-72.
39. Vernieri C, Casola S, Foiani M, Pietrantonio F, de Braud F, Longo V. Targeting cancer metabolism: dietary and pharmacologic interventions. Cancer Discov. 2016;6:1315-33.
40. Zhao H, Yang L, Baddour J, et al. Tumor microenvironment derived exosomes pleiotropically modulate cancer cell metabolism. Elife. 2016;5:e10250.
41. Talbott HE, Mascharak S, Griffin M, Wan DC, Longaker MT. Wound healing, fibroblast heterogeneity, and fibrosis. Cell Stem Cell. 2022;29:1161-80.
42. Plikus MV, Wang X, Sinha S, et al. Fibroblasts: origins, definitions, and functions in health and disease. Cell. 2021;184:3852-72.
43. Oh EJ, Gangadaran P, Rajendran RL, et al. Extracellular vesicles derived from fibroblasts promote wound healing by optimizing fibroblast and endothelial cellular functions. Stem Cells. 2021;39:266-79.
44. Xia W, Li M, Jiang X, et al. Young fibroblast-derived exosomal microRNA-125b transfers beneficial effects on aged cutaneous wound healing. J Nanobiotechnology. 2022;20:144.
46. Hu JL, Wang W, Lan XL, et al. CAFs secreted exosomes promote metastasis and chemotherapy resistance by enhancing cell stemness and epithelial-mesenchymal transition in colorectal cancer. Mol Cancer. 2019;18:91.
47. Wang X, Qin X, Yan M, et al. Loss of exosomal miR-3188 in cancer-associated fibroblasts contributes to HNC progression. J Exp Clin Cancer Res. 2019;38:151.
48. Wortzel I, Dror S, Kenific CM, Lyden D. Exosome-mediated metastasis: communication from a distance. Dev Cell. 2019;49:347-60.
49. Shi L, Zhu W, Huang Y, et al. Cancer-associated fibroblast-derived exosomal microRNA-20a suppresses the PTEN/PI3K-AKT pathway to promote the progression and chemoresistance of non-small cell lung cancer. Clin Transl Med. 2022;12:e989.
50. Chen B, Sang Y, Song X, et al. Exosomal miR-500a-5p derived from cancer-associated fibroblasts promotes breast cancer cell proliferation and metastasis through targeting USP28. Theranostics. 2021;11:3932-47.
51. Xu G, Zhang B, Ye J, et al. Exosomal miRNA-139 in cancer-associated fibroblasts inhibits gastric cancer progression by repressing MMP11 expression. Int J Biol Sci. 2019;15:2320-9.
52. Yugawa K, Yoshizumi T, Mano Y, et al. Cancer-associated fibroblasts promote hepatocellular carcinoma progression through downregulation of exosomal miR-150-3p. Eur J Surg Oncol. 2021;47:384-93.
53. Zhang Z, Li X, Sun W, et al. Loss of exosomal miR-320a from cancer-associated fibroblasts contributes to HCC proliferation and metastasis. Cancer Lett. 2017;397:33-42.
54. Valastyan S, Weinberg RA. Tumor metastasis: molecular insights and evolving paradigms. Cell. 2011;147:275-92.
55. Fares J, Fares MY, Khachfe HH, Salhab HA, Fares Y. Molecular principles of metastasis: a hallmark of cancer revisited. Signal Transduct Target Ther. 2020;5:28.
56. Li YY, Tao YW, Gao S, et al. Cancer-associated fibroblasts contribute to oral cancer cells proliferation and metastasis via exosome-mediated paracrine miR-34a-5p. EBioMedicine. 2018;36:209-20.
57. Miyoshi A, Kitajima Y, Kido S, et al. Snail accelerates cancer invasion by upregulating MMP expression and is associated with poor prognosis of hepatocellular carcinoma. Br J Cancer. 2005;92:252-8.
58. Wu HJ, Hao M, Yeo SK, Guan JL. FAK signaling in cancer-associated fibroblasts promotes breast cancer cell migration and metastasis by exosomal miRNAs-mediated intercellular communication. Oncogene. 2020;39:2539-49.
59. Al-Raimi HAI, Kong J, Ran Y, et al. Extracellular vesicles from carcinoma-associated fibroblasts promote EMT of salivary adenoid cystic carcinoma via IL-6. Arch Med Res. 2023;54:27-36.
60. Benavente S. Remodeling the tumor microenvironment to overcome treatment resistance in HPV-negative head and neck cancer. Cancer Drug Resist. 2023;6:291-313.
61. Feng R, Li Z, Ge G, Wang C, Jia Y, Ouyang J. Cancer-associated fibroblast-derived extracellular vesicles mediate immune escape of bladder cancer via PD-L1/PD-1 expression. Endocr Metab Immune Disord Drug Targets. 2023;23:1410-20.
62. Zhao Q, Huang L, Qin G, et al. Cancer-associated fibroblasts induce monocytic myeloid-derived suppressor cell generation via IL-6/exosomal miR-21-activated STAT3 signaling to promote cisplatin resistance in esophageal squamous cell carcinoma. Cancer Lett. 2021;518:35-48.
63. Wang T, Zhang H. Exploring the roles and molecular mechanisms of RNA binding proteins in the sorting of noncoding RNAs into exosomes during tumor progression. J Adv Res. 2024;65:105-23.
64. Wang WZ, Cao X, Bian L, et al. Analysis of mRNA-miRNA interaction network reveals the role of CAFs-derived exosomes in the immune regulation of oral squamous cell carcinoma. BMC Cancer. 2023;23:591.
65. Jain RK. Normalization of tumor vasculature: an emerging concept in antiangiogenic therapy. Science. 2005;307:58-62.
66. Zhang J, Pan Y, Jin L, Yang H, Cao P. Exosomal-miR-522-3p derived from cancer-associated fibroblasts accelerates tumor metastasis and angiogenesis via repression bone morphogenetic protein 5 in colorectal cancer. J Gastroenterol Hepatol. 2024;39:107-20.
67. Wu C, Li D, Cheng X, Gu H, Qian Y, Feng L. Downregulation of cancer-associated fibroblast exosome-derived miR-29b-1-5p restrains vasculogenic mimicry and apoptosis while accelerating migration and invasion of gastric cancer cells via immunoglobulin domain-containing 1/zonula occluden-1 axis. Cell Cycle. 2023;22:1807-26.
68. Sun M, Wang X, Shou Y, et al. Cancer-associated fibroblast-derived exosome microRNA-21 promotes angiogenesis in multiple myeloma. Sci Rep. 2023;13:9671.
69. Chen C, Yang C, Tian X, et al. Downregulation of miR-100-5p in cancer-associated fibroblast-derived exosomes facilitates lymphangiogenesis in esophageal squamous cell carcinoma. Cancer Med. 2023;12:14468-83.
70. Melo SA, Luecke LB, Kahlert C, et al. Glypican-1 identifies cancer exosomes and detects early pancreatic cancer. Nature. 2015;523:177-82.
71. Tian F, Zhang S, Liu C, et al. Protein analysis of extracellular vesicles to monitor and predict therapeutic response in metastatic breast cancer. Nat Commun. 2021;12:2536.
72. Das S, Lyon CJ, Hu T. A panorama of extracellular vesicle applications: from biomarker detection to therapeutics. ACS Nano. 2024;18:9784-97.
73. Wu Q, Fu S, Xiao H, et al. Advances in extracellular vesicle nanotechnology for precision theranostics. Adv Sci. 2023;10:e2204814.
74. Muskan M, Abeysinghe P, Cecchin R, Branscome H, Morris KV, Kashanchi F. Therapeutic potential of RNA-enriched extracellular vesicles: the next generation in RNA delivery via biogenic nanoparticles. Mol Ther. 2024;32:2939-49.
75. Santos NL, Bustos SO, Reis PP, Chammas R, Andrade LNS. Extracellular vesicle-packaged miR-195-5p sensitizes melanoma to targeted therapy with kinase inhibitors. Cells. 2023;12:1317.
76. Katakowski M, Buller B, Zheng X, et al. Exosomes from marrow stromal cells expressing miR-146b inhibit glioma growth. Cancer Lett. 2013;335:201-4.
77. Zhang Y, Li L, Yu J, et al. Microvesicle-mediated delivery of transforming growth factor β1 siRNA for the suppression of tumor growth in mice. Biomaterials. 2014;35:4390-400.
78. Xue X, Wang X, Pang M, et al. An exosomal strategy for targeting cancer-associated fibroblasts mediated tumors desmoplastic microenvironments. J Nanobiotechnology. 2024;22:196.
79. Hoshino A, Costa-Silva B, Shen TL, et al. Tumour exosome integrins determine organotropic metastasis. Nature. 2015;527:329-35.
80. Zhan Q, Liu B, Situ X, et al. New insights into the correlations between circulating tumor cells and target organ metastasis. Signal Transduct Target Ther. 2023;8:465.
81. Hade MD, Suire CN, Mossell J, Suo Z. Extracellular vesicles: emerging frontiers in wound healing. Med Res Rev. 2022;42:2102-25.
82. Romano M, Zendrini A, Paolini L, et al. 2 - extracellular vesicles in regenerative medicine. In: Rossi F, Rainer A, Editors. Nanomaterials for Theranostics and Tissue Engineering. Elsevier; 2020. pp. 29-58. Available from: https://www.sciencedirect.com/science/article/abs/pii/B9780128178386000024. [Last accessed on 6 Jan 2025].
83. Welsh JA, Goberdhan DC, O'Driscoll L, Théry C, Witwer KW. MISEV2023: an updated guide to EV research and applications. J Extracell Vesicles. 2024;13:e12416.
84. Andaloussi S, Lakhal S, Mäger I, Wood MJ. Exosomes for targeted siRNA delivery across biological barriers. Adv Drug Deliv Rev. 2013;65:391-7.
85. Zhang L, Wu T, Shan Y, et al. Therapeutic reversal of Huntington’s disease by in vivo self-assembled siRNAs. Brain. 2021;144:3421-35.
86. Bashyal S, Thapa C, Lee S. Recent progresses in exosome-based systems for targeted drug delivery to the brain. J Control Release. 2022;348:723-44.
Cite This Article
How to Cite
Xie, J.; Lin, X.; Deng, X.; Tang, H.; Zou, Y.; Chen, W.; Xie, X. Cancer-associated fibroblast-derived extracellular vesicles: regulators and therapeutic targets in the tumor microenvironment. Cancer. Drug. Resist. 2025, 8, 2. http://dx.doi.org/10.20517/cdr.2024.152
Download Citation
Export Citation File:
Type of Import
Tips on Downloading Citation
Citation Manager File Format
Type of Import
Direct Import: When the Direct Import option is selected (the default state), a dialogue box will give you the option to Save or Open the downloaded citation data. Choosing Open will either launch your citation manager or give you a choice of applications with which to use the metadata. The Save option saves the file locally for later use.
Indirect Import: When the Indirect Import option is selected, the metadata is displayed and may be copied and pasted as needed.
About This Article
Special Issue
Copyright
Data & Comments
Data
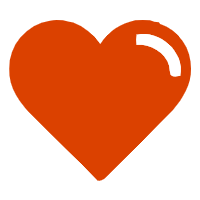
Comments
Comments must be written in English. Spam, offensive content, impersonation, and private information will not be permitted. If any comment is reported and identified as inappropriate content by OAE staff, the comment will be removed without notice. If you have any queries or need any help, please contact us at support@oaepublish.com.