Coexistence of ferroelectric and ferrielectric phases in ultrathin antiferroelectric PbZrO3 thin films
Abstract
Whereas ferroelectricity may vanish in ultra-thin ferroelectric films, it is expected to emerge in ultra-thin antiferroelectric films, sparking people’s interest in using antiferroelectric materials as an alternative to ferroelectric ones for high-density data storage applications. Lead Zirconate (PbZrO3, PZO) is considered the prototype material for antiferroelectricity, and indeed, previous studies indicated that nanoscale PZO films exhibit ferroelectricity. The understanding of such phenomena from the microstructure aspect is crucial but still lacking. In this study, we fabricated a PZO film with thicknesses varying from 5 to 80 nm. Using Piezoresponse Force Microscopy, we discovered that the film displayed a transition from antiferroelectric behavior in the thicker areas to ferroelectric behavior in the thinner ones, with a critical thickness between 10 and 15 nm. In this critical thickness range, a 12 nm PZO thin film was chosen for further study using aberration-corrected scanning transmission electron microscopy. The investigation showed that the film comprises both ferroelectric and ferrielectric phases. The ferroelectric phase is characterized by polarization along the [011]pc projection direction. The positions of Pb, Zr, and O were determined using the integrated differential phase contrast method. This allowed us to ascertain that the ferroelectric PZO unit cell is half the size of that in the antiferroelectric phase on the ab plane. The observed unit cell is different from the electric field-induced ferroelectric rhombohedral phases. Additionally, we identified a ferrielectric phase with a unique up-up-zero-zero (↑↑··) dipole configuration. The finding is crucial for understanding the performance of ultrathin antiferroelectric thin films and the subsequent design and development of antiferroelectric devices.
Keywords
INTRODUCTION
Antiferroelectric (AFE) materials feature antiparallel electric dipoles that can be realigned into a parallel arrangement when subjected to a sufficiently high electric field[1-4]. This realignment can be viewed as a field-induced phase transition from AFE to ferroelectric (FE), a process that is marked by significant charge storage, volume expansion, and a change in temperature (electrocaloric effect)[5-8]. Such properties make these materials suitable for use in a variety of electronic devices, including capacitors with high energy/power density, actuators capable of large strains, solid-state refrigeration, and advanced thermal switches[6,9-12]. For most applications, antiferroelectric materials are best utilized when integrated into electronic devices as thin films. Moreover, the demand for smaller, higher-performance electronic devices that can be actuated with smaller voltages has led to the preparation of even thinner films. Yet, as antiferroelectric films are made thinner, it is often observed that their ground state evolves from antipolar to polar[13]. This represents both a challenge for applications relying on antiferroelectric switching, and an opportunity for applications, such as memory devices and ferroelectric tunnel junctions, where very thin ferroelectrics are desirable. In either case, identifying the critical thickness for the AFE-FE transition and determining how the structure evolves from one to the other (whether abruptly or through an intermediate range of phase coexistence) is essential.
PbZrO3 (PZO) was the first antiferroelectric material discovered and is considered the archetype[2]. It is distinguished by an up-up-down-down (↑↑↓↓) dipole pattern within a unit cell[3]. In antiferroelectric PZO thin films or even in single crystals, various ferrielectric phases, which show antiparallel but uncompensated electric dipoles, have been identified to exist[14-20]. When the thickness of PZO thin films is reduced to the nanometer scale, they are reported to transition to a ferroelectric rhombohedral phase[13]. Since ferroelectric materials often suffer from reduced or completely lost polarization at the heterointerface due to depolarization effects[21,22], using antiferroelectric materials instead of ferroelectric ones in ultra-high-density information storage could offer a promising alternative. However, these ultrathin antiferroelectric films have not been extensively explored, and their ferroelectric properties have yet to be verified from a structural standpoint. The lack of this understanding has impeded their use in electronic devices.
In this work, we have fabricated a PZO thin film with thicknesses ranging from 5 to 80 nm and an ultrathin PZO film of about 12 nm. Combining X-ray diffraction (XRD), scanning probe microscopy and aberration-corrected transmission electron microscopy (TEM), we investigated the ferroelectricity and the phases in PZO epitaxial films, including one with a thickness gradient such that, within the same sample, the thick side is antiferroelectric and the thin end is ferroelectric.
MATERIALS AND METHODS
Thin film fabrication: Using a Pulsed Laser Deposition (PLD) system with a KrF excimer laser (COMPex 102, Lambda Physik, λ = 248 nm), PZO thin films of about 12 nm were grown on SrTiO3 (STO) (001) substrates (CrysTec GmbH) covered by SrRuO3 (SRO) bottom electrodes. Before deposition, the base pressure of the chamber was brought down to 3.0 × 10-3 mTorr. Both PZO and SRO layers were deposited under an oxygen pressure of 100 mTorr, with a laser fluence of 2.0-2.5 J/cm2 at a laser repetition rate of
X-ray diffraction (XRD) measurements: High-resolution θ - 2θ scans and reciprocal space maps (RSMs) were measured using a four-circle diffractometer equipped with 2 × Ge(220) monochromator (Malvern PANalytical X’pert Pro MRD, Cu Kα1, λ = 1.5406 Å). The local measurements at different X-positions across the sample were made by using a narrow divergence slit of 1/4°. RSMs were measured in the region where pseudocubic (
Scanning Probe Microscopy investigation (SPM): Atomic force microscopy (AFM) and piezoresponse force microscopy (PFM) experiments were carried out using a scanning probe microscopy (SPM) system (MFP-3D Classic, Asylum Research, Santa Barbara, CA, USA) in tapping and dual-AC resonance tracking mode, respectively. The PPP-EFM probe (Nanosensors, Neuchâtel, Switzerland) was used for acquiring topographic images and phase and amplitude curves.
Transmission Electron Microscopy (TEM) investigation: Cross-sectional TEM specimens of PZO thin films oriented along the pseudocubic [100] direction were prepared by slicing, gluing, polishing, and finally ion milling. An FEI Tecnai F20 Transmission Electron Microscope (operated at 200 kV) was employed for the low magnification morphology observation of the thin film. Atomic-scale scanning TEM high angle annular dark field (STEM-HAADF) and integrated differential phase contrast (iDPC) images were acquired using a Thermo Fisher Themis-Z Double-corrected 60-300 kV scanning/transmission electron microscope
RESULTS AND DISCUSSION
Figure 1A shows the XRD θ - 2θ scan for a PZO thin film whose thickness changes from 5 to 80 nm with a gradient of ~7.5 nm/mm. Thickness at different positions was estimated from the full width at half maximum (FWHM) of the PZO film reflections in the 2theta scan by applying the Scherrer equation, assuming the instrumental width from the substrate (002) peak. Two peaks for PZO can be seen in the
Figure 1. (A) XRD diffraction pattern of a thickness-gradient sample (PZO/SRO/STO) ranging from 5 to 80 nm thick with a slope of ~7.5 nm/mm. The sample is 10 mm in length. The pattern exhibits a gradual shift of the PZO peaks, from the two peaks of the orthorhombic AFE phase dominant at higher thickness towards convolution with an emerging third peak with an intermediate lattice parameter, corresponding to the FE phase that becomes dominant in the thin region. (B) The lattice parameter of the PZO shifts with its thickness. (C-E) Reciprocal Space Map measurements of a gradient sample (PZO/SRO/STO) with a slope for the PZO of ~7.5 nm/mm, 80, 45 and 9 nm thick. The top signal close to STO corresponds to the SRO (
To determine whether the film exhibits ferroelectric or antiferroelectric characteristics, PFM measurements were carried out. Figure 2A and B presents the PFM phase and amplitude curves for the 80 nm PZO region. The phase curve shows a 180° difference between the positive and negative voltages, attributable to the field-induced ferroelectric states. The amplitude curve exhibits four peaks (indicated by red arrows), and at voltages below the critical voltage for the AFE to FE transition, the amplitude signal is very small, suggesting antiferroelectric characteristics[28,29]. The piezoresponse curve was calculated using Amplitude × cos (Phase), and is shown in Supplementary Figure 1. The double hysteresis loop further suggests the antiferroelectric characteristics. Two additional amplitude curves are displayed in Supplementary Figure 2, where small amplitudes around zero voltage and the characteristic four amplitude peaks are visible. Figure 2C and D displays the PFM phase and amplitude response curves obtained from a region estimated to be 12.5 nm. The 180° phase difference of the hysteresis loop and the butterfly-like amplitude behaviors indicate the ferroelectric characteristics of the 12.5 nm PZO. The piezoresponse curve of the 12.5 nm PZO is shown in Supplementary Figure 3. The single hysteresis loop further indicates the ferroelectric characteristics. Phase and amplitude curves of 5 nm PZO are displayed in Supplementary Figure 4; although these curves are noisy, they still show the ferroelectric characteristics.
Figure 2. PFM phase (A) and amplitude (B) curves from 80 nm PZO region (voltage on-mode). PFM phase (C) and amplitude (D) curves (voltage off-mode) acquired from the PZO thin film of about 12.5 nm thick.
To investigate the microstructure of PZO at the AFE-FE transition critical thickness, we have prepared a PZO thin film with a constant thickness of 12 nm, grown under the same conditions as the gradient-thickness sample. Figure 3A presents the XRD θ - 2θ profile of this sample. Peaks corresponding to the STO substrate, SRO buffer layer and PZO are marked with black, blue and purple arrows, respectively. The broad peak for the PZO (002)pc (pc: pseudo-cubic) indicates a variation in the out-of-plane lattice constants. The peak position corresponds to 2 of 43.6°, and gives rise to an out-of-plane (001)pc lattice spacing of 4.148 Å, consistent with the pseudo-cubic lattice constant (
Figure 3. (A) XRD θ - 2θ profiles around STO (002) diffraction obtained from a 12 nm PZO thin film that was grown on SRO (about
Then, we further examined the microstructure of the 12 nm PZO thin film using aberration-corrected STEM. A high-resolution STEM-HAADF image showing the PZO (top) and SRO (bottom) layers is presented in Figure 4A. At the PZO/SRO interface, dislocations with Burgers vector of a[100] were revealed most frequently, as outlined using Burgers circuit (white lines) and marked by white arrows, while occasionally ½a[101] type dislocations (not shown here) were also observed. This is not quite the same as the primarily ½a[101] dislocations reported for an 8 nm thick PZO thin film[13]. The δPb map (yellow arrows) was superimposed on the HAADF image, where parallel instead of antiparallel δPb is evident, with Pb displacement pointing toward [10
Figure 4. (A) A STEM-HAADF image superimposed by δPb map (yellow arrows) showing the ferroelectric phase observed in PZO ultrathin film (12 nm). (B) GPA of in-plane strain (εxx) map of HAADF image in (A). (C) FFT of the PZO layer in (A). (D) An iDPC image showing the oxygen positions in the ferroelectric phase observed in PZO ultrathin films. A ferroelectric unit cell is outlined in panel (D).
The GPA in-plane lattice strain map shown in Figure 4B suggests a uniform in-plane strain of about 6% with respect to SRO and an in-plane lattice constant of 0.414 nm in PZO, in contrast with the antiferroelectric or ferrielectric periodic modulations[14,31]. A Fast Fourier transition (FFT) of the PZO layer is shown in Figure 4C, from where superlattice diffraction spots at ½(110) positions were evident as indicated by a yellow arrow, suggesting the formation of superlattice concerning cubic perovskite structure. Such a superlattice diffraction was considered to be a ferroelectric rhombohedral phase[13].
To delve into the structure of the ferroelectric phase, iDPC experiments [Figure 4D] were conducted, which have been demonstrated to effectively visualize oxygen in perovskite oxides[32,33]. In Figure 4D, Pb (grey circles), Zr (green circles) and O (red circles) positions are superimposed on the high-resolution iDPC image. The distortion of oxygen octahedra can be determined, as outlined by white lines. A clear difference in the distortion patterns of the neighboring oxygen octahedra can be identified. This variation is also noticeable in the undulating patterns of oxygen atom chains within the ZrO2 layer - with chains alternating between higher and lower positions horizontally, and left and right positions vertically. These patterns are highlighted by yellow polylines connecting the red circles (O) in Figure 4D. The oxygen atom columns appear round, indicating that the adjacent oxygen octahedra along the observation direction rotate in the same direction, or in other words, exhibit in-phase rotation (glazer notation of c+). This is different from the antiphase rotation (c-) of the rhombohedral ferroelectric phase with the R3c space group[34]. It is also inconsistent with the ferroelectric rhombohedral phase with an R3m space group, which does not show oxygen octahedral distortion[35]. Based on the oxygen position characteristics, a projected 2D unit cell can be determined to be twice that of pseudo-cubic perovskite (as outlined by blue square), and half of the ab plane of the orthorhombic antiferroelectric PZO. The determined unit cell is consistent with the superlattice diffraction spots observed in the FFT image in Figure 4C. If we assume that the differences between the ferroelectric and antiferroelectric phases are solely due to Pb displacement and oxygen octahedral rotation, it would imply that the ferroelectric phase possesses orthorhombic symmetry, akin to antiferroelectric PZO, but with a unit cell size reduced by half. To the best of our knowledge, this phase has not been previously reported in PZO.
In addition to the ferroelectric phase, a ferrielectric phase with the dipole of ↑↑·· (with half of the δPb negligible and marked as “·”) was also unraveled in the 12 nm thick PZO thin film, as displayed in Figure 5A and marked by Region I. The in-plane lattice strain map, generated using GPA and presented in
Figure 5. (A) A STEM-HAADF image superimposed by δPb map (yellow arrows) showing the coexistence of ferroelectric and ferrielectric phases in PZO ultrathin films. (B) The GPA in-plane lattice map of (A). (C) Fast Fourier transition of the PZO layer containing region I in (A).
Then, we tried to figure out the reason for the formation of such ferroelectric and ferrielectric phases. For PZO, it is well known that the electric field can force the AFE to FE transition. Previous first-principles simulation results suggest strain can also cause the AFE to FE phase transition[37], while previous experimental results on the PZO thin films suggested the strain in the PZO layer has stabilized the ferroelectric rhombohedral phase in the PZO of 8 nm thick[13]. In this work, we have analyzed the strain state in the thin film precisely but did not find an obvious difference in the ferroelectric region.
A few factors may contribute to the ferroelectric and ferrielectric phases. The first one is the built-in field caused by the energy-band-mismatch at the PZO/SRO interface. Our PFM results indicate the existence of a built-in bias, as illustrated in the Amplitude curve of Figure 2B. The bias voltage is of the order of 0.2-0.3 V which, divided by the thickness of a 10 nm film, translates into a field of 200-300 kV/cm. It, in principle, favors a ferroelectric transition.
The second one is the interfacial misfit dislocations caused by the flexoelectric field. Edge dislocations have been proved to cause a strain gradient along the direction perpendicular to the Burges vector[32]. The flexocoupling coefficient (f) of PZO was measured to be around 3 V at room temperature[38], while the maximum strain gradient (
Surface effects might also play a role in the phase transition. The observation of ferroelectricity in ultrathin antiferroelectric NaNbO3 membranes suggests that distortions/chemical environments beginning at the surface are responsible for driving the ferroelectric phase[39]. Here, we have conducted TEM experiments on the surface of PZO thin films. Our TEM results for antiferroelectric thin film [Supplementary Figure 5] suggest that the top five unit cells (2 nm) are polar, suggesting the surfaces may inherently help stabilize ferroelectricity in very thin films, as predicted by Mani et al.[40]. Yet, the surface polar layer is only a few unit cells, indicating the surface effect alone may not be sufficient to induce the ferroelectric phase in films that are more than 10 nm thick.
Furthermore, the rotation of oxygen octahedra from the substrate can spread into the thin film, prompting a phase transition. For instance, in thin films of the multiferroic material BiFeO3, oxygen octahedral rotations from the substrate have been shown to transfer to the BiFeO3 layer[41]. In the ferroelectric phase observed in this study, when viewed along the c-axis (as shown in Figure 4), the oxygen octahedra exhibiting in-phase rotation corresponds to the Glazer notation of c+, which is the same as for the SRO electrode. The pattern of oxygen octahedral rotation in SRO is therefore replicated by the PZO, suggesting that octahedral coupling may facilitate the formation of the ferroelectric phase. However, previous studies on BiFeO3 thin films grown on NdGaO3 substrates indicate that only the first three unit cells are influenced by the substrate’s oxygen octahedral coupling[41]. Consequently, we believe that coupling oxygen octahedral rotation may help but is unlikely to be the sole factor contributing to the observed ferroelectric phase.
Based on the analysis presented, it is impossible to single out a unique factor - whether it be the built-in field, the flexoelectric field induced by dislocations, surface effects, or oxygen octahedral rotations - for inducing the ferroelectric phase in the 10 nm films. Rather, it seems likely to be a combination of these influences.
CONCLUSIONS
PZO thin films with a thickness ranging from 5 to 80 nm were fabricated and a transition from an AFE to FE phase was confirmed through the PFM phase and amplitude curves, suggesting the potential application of ultrathin PZO in memory devices for data storage. Using aberration-corrected STEM-HAADF and iDPC imaging techniques, atomic resolution images were captured from a 12 nm PZO thin film. It revealed not only Pb and Zr but also O positions, thereby confirming a ferroelectric phase with polarization along [101]pc projection direction. This is an important result because it indicates that the ferroelectric phase of ultra-thin PZO is NOT the same as the field-induced ferroelectric rhombohedral phase of bulk PZO. In fact, it is closer to the “ideal” of an AFE to FE phase transition where the sub-lattice polarizations become parallel to each other by changing the orientation of just one of them, without changing the crystal class[42]. Additionally, a ferrielectric phase characterized by a dipole pattern of ↑↑·· was identified, providing a natural bridging step between the perfectly antipolar and perfectly polar states.
DECLARATIONS
Acknowledgments
The authors are grateful for the scientific and technical support from the Australian Centre for Microscopy and Microanalysis (ACMM) as well as the Microscopy Australia node at the University of Sydney.
Authors’ contributions
Experimental design, PFM measurements and data analysis: Catalan G, Liu Y
Thin film fabrication: Liu Y, Uriach R, Roque JMC
XRD measurements and data analysis: Santiso J, Liu Y, Pesquera D, Uriach R, Catalan G
TEM experiment and data analysis: Liu Y, Niu R, Cairney JM, Liao X, Catalan G
Manuscript writing and revision: Liu Y
Supervision: Catalan G, Arbiol J, Liao X, Cairney JM
All authors revised the manuscript.
Availability of data and materials
The data that support the findings of this study are available from the corresponding author upon reasonable request.
Financial support and sponsorship
This project has received funding from the European Union’s Horizon 2020 research and innovation program under Grant Agreement No. 766726 (TSAR). Liu Y acknowledges the BIST Postdoctoral Fellowship Programme (PROBIST) funded by the European Union’s Horizon 2020 research and innovation programme under the Marie Sklodowska-Curie Grant Agreement No. 754510. Pesquera D acknowledges funding from the "la Caixa" Foundation fellowship (ID 100010434). Liao X is supported by the Australian Research Council Discovery Project DP190101155. ICN2 acknowledges funding from Generalitat de Catalunya 2021SGR00457. ICN2 is supported by the Severo Ochoa program from Spanish MCIN/AEI (Grant No.: CEX2021-001214-S) and is funded by the CERCA Programme/Generalitat de Catalunya.
Conflicts of interest
Liao X is Executive Editor of the journal Microstructures, while the other authors have declared that they have no conflicts of interest.
Ethical approval and consent to participate
Not applicable.
Consent for publication
Not applicable.
Copyright
© The Author(s) 2024.
Supplementary Materials
REFERENCES
2. Shirane G, Sawaguchi E, Takagi Y. Dielectric properties of lead zirconate. Phys Rev 1951;84:476-81.
3. Sawaguchi E, Maniwa H, Hoshino S. Antiferroelectric structure of lead zirconate. Phys Rev 1951;83:1078.
4. Randall CA, Fan Z, Reaney I, Chen L, Trolier-mckinstry S. Antiferroelectrics: history, fundamentals, crystal chemistry, crystal structures, size effects, and applications. J Am Ceram Soc 2021;104:3775-810.
5. Liu Z, Lu T, Ye J, et al. Antiferroelectrics for energy storage applications: a review. Adv Mater Technol 2018;3:1800111.
6. Pan WY, Dam CQ, Zhang QM, Cross LE. Large displacement transducers based on electric field forced phase transitions in the tetragonal (Pb0.97La0.02)(Ti,Zr,Sn)O3 family of ceramics. J Appl Phys 1989;66:6014-23.
7. Pirc R, Rožič B, Koruza J, Malič B, Kutnjak Z. Negative electrocaloric effect in antiferroelectric PbZrO3. EPL 2014;107:17002.
8. Tan X, Ma C, Frederick J, Beckman S, Webber KG. The antiferroelectric ↔ ferroelectric phase transition in lead-containing and lead-free perovskite ceramics. J Am Ceram Soc 2011;94:4091-107.
9. Liu C, Si Y, Zhang H, et al. Low voltage-driven high-performance thermal switching in antiferroelectric PbZrO3 thin films. Science 2023;382:1265-9.
10. Si Y, Zhang T, Liu C, et al. Antiferroelectric oxide thin-films: fundamentals, properties, and applications. Prog Mater Sci 2024;142:101231.
11. Chauhan A, Patel S, Vaish R, Bowen CR. Anti-ferroelectric ceramics for high energy density capacitors. Materials 2015;8:8009-31.
12. Liu G, Chen L, Qi H. Energy storage properties of NaNbO3-based lead-free superparaelectrics with large antiferrodistortion. Microstructures 2023;3:2023009.
13. Roy Chaudhuri A, Arredondo M, Hähnel A, et al. Epitaxial strain stabilization of a ferroelectric phase in PbZrO3 thin films. Phys Rev B 2011;84:054112.
14. Liu Y, Niu R, Majchrowski A, et al. Translational boundaries as incipient ferrielectric domains in antiferroelectric PbZrO3. Phys Rev Lett 2023;130:216801.
15. Yao Y, Naden A, Tian M, et al. Ferrielectricity in the archetypal antiferroelectric, PbZrO3. Adv Mater 2023;35:e2206541.
16. Jiang RJ, Cao Y, Geng WR, et al. Atomic insight into the successive antiferroelectric-ferroelectric phase transition in antiferroelectric oxides. Nano Lett 2023;23:1522-9.
17. Fu Z, Chen X, Li Z, et al. Unveiling the ferrielectric nature of PbZrO3-based antiferroelectric materials. Nat Commun 2020;11:3809.
18. Ma T, Fan Z, Xu B, et al. Uncompensated polarization in incommensurate modulations of perovskite antiferroelectrics. Phys Rev Lett 2019;123:217602.
19. Burkovsky RG, Lityagin GA, Ganzha AE, et al. Field-induced heterophase state in PbZrO3 thin films. Phys Rev B 2022;105:125409.
20. Wei XK, Dunin-Borkowski RE, Mayer J. Structural phase transition and in-situ energy storage pathway in nonpolar materials: a review. Materials 2021;14:7854.
21. Jia CL, Nagarajan V, He JQ, et al. Unit-cell scale mapping of ferroelectricity and tetragonality in epitaxial ultrathin ferroelectric films. Nat Mater 2007;6:64-9.
22. Chisholm MF, Luo W, Oxley MP, Pantelides ST, Lee HN. Atomic-scale compensation phenomena at polar interfaces. Phys Rev Lett 2010;105:197602.
23. Hytch MJ, Snoeck E, Kilaas R. Quantitative measurement of displacement and strain fields from HREM micrographs. Ultramicroscopy 1998;74:131-46.
24. Tang YL, Zhu YL, Ma XL, et al. Observation of a periodic array of flux-closure quadrants in strained ferroelectric PbTiO3 films. Science 2015;348:547-51.
25. Liu Y, Wang YJ, Zhu YL, et al. Large scale two-dimensional flux-closure domain arrays in oxide multilayers and their controlled growth. Nano Lett 2017;17:7258-66.
26. Liu Y, Cui X, Niu R, et al. Giant room temperature compression and bending in ferroelectric oxide pillars. Nat Commun 2022;13:335.
27. Nord M, Vullum PE, MacLaren I, Tybell T, Holmestad R. Atomap: a new software tool for the automated analysis of atomic resolution images using two-dimensional Gaussian fitting. Adv Struct Chem Imaging 2017;3:9.
28. Boldyreva K, Bao D, Le Rhun G, Pintilie L, Alexe M, Hesse D. Microstructure and electrical properties of (120)O-oriented and of (001)O-oriented epitaxial antiferroelectric PbZrO3 thin films on (100) SrTiO3 substrates covered with different oxide bottom electrodes. J Appl Phys 2007;102:044111.
29. Lu H, Glinsek S, Buragohain P, Defay E, Iñiguez J, Gruverman A. Probing antiferroelectric-ferroelectric phase transitions in PbZrO3 capacitors by piezoresponse force microscopy. Adv Funct Mater 2020;30:2003622.
30. Corker DL, Glazer AM, Dec J, Roleder K, Whatmore RW. A re-investigation of the crystal structure of the perovskite PbZrO3 by
31. Saeed U, Pesquera D, Liu Y, et al. Switching dynamics and improved efficiency of free-standing antiferroelectric capacitors. Adv Electron Mater 2024:2400102.
32. Liu Y, Niu RM, Moss SD, Finkel P, Liao XZ, Cairney JM. Atomic coordinates and polarization map around a pair of ½a[01
33. Cabral MJ, Chen Z, Liao X. Scanning transmission electron microscopy for advanced characterization of ferroic materials. Microstructures 2023;3:2023040.
34. Dmowski W, Egami T, Farber L, et al. Structure of Pb(Zr,Ti)O3 near the morphotropic phase boundary. AIP Conf Proc 2001;582:33-44.
35. Joseph J, Vimala TM, Sivasubramanian V, Murthy VRK. Structural investigations on Pb(ZrxT1-x)O3 solid solutions using the X-ray rietveld method. J Mater Sci 2000;35:1571-5.
36. Tolédano P, Guennou M. Theory of antiferroelectric phase transitions. Phys Rev B 2016;94:014107.
37. Reyes-Lillo SE, Rabe KM. Antiferroelectricity and ferroelectricity in epitaxially strained PbZrO3 from first principles. Phys Rev B 2013;88:180102(R).
38. Vales-Castro P, Roleder K, Zhao L, Li J, Kajewski D, Catalan G. Flexoelectricity in antiferroelectrics. Appl Phys Lett 2018;113:132903.
39. Xu R, Crust KJ, Harbola V, et al. Size-induced ferroelectricity in antiferroelectric oxide membranes. Adv Mater 2023;35:e2210562.
40. Mani BK, Chang CM, Lisenkov S, Ponomareva I. Critical thickness for antiferroelectricity in PbZrO3. Phys Rev Lett 2015;115:097601.
41. Han MJ, Wang YJ, Ma DS, et al. Coexistence of rhombohedral and orthorhombic phases in ultrathin BiFeO3 films driven by interfacial oxygen octahedral coupling. Acta Mater 2018;145:220-6.
Cite This Article
How to Cite
Liu, Y.; Niu R.; Uriach R.; Pesquera D.; Roque J. M. C.; Santiso J.; Cairney J. M.; Liao X.; Arbiol J.; Catalan G. Coexistence of ferroelectric and ferrielectric phases in ultrathin antiferroelectric PbZrO3 thin films. Microstructures. 2024, 4, 2024045. http://dx.doi.org/10.20517/microstructures.2024.12
Download Citation
Export Citation File:
Type of Import
Tips on Downloading Citation
Citation Manager File Format
Type of Import
Direct Import: When the Direct Import option is selected (the default state), a dialogue box will give you the option to Save or Open the downloaded citation data. Choosing Open will either launch your citation manager or give you a choice of applications with which to use the metadata. The Save option saves the file locally for later use.
Indirect Import: When the Indirect Import option is selected, the metadata is displayed and may be copied and pasted as needed.
About This Article
Special Issue
Copyright
Data & Comments
Data
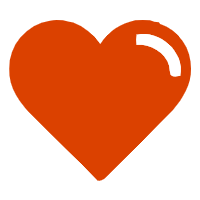
Comments
Comments must be written in English. Spam, offensive content, impersonation, and private information will not be permitted. If any comment is reported and identified as inappropriate content by OAE staff, the comment will be removed without notice. If you have any queries or need any help, please contact us at support@oaepublish.com.