Intercellular transfer of multidrug resistance mediated by extracellular vesicles
Abstract
Multidrug resistance (MDR) poses a formidable obstacle in cancer treatment, enabling cancer cells to evade the cytotoxic effects of chemotherapeutic drugs through various mechanisms. These mechanisms include intrinsic resistance, which is present prior to treatment, and acquired resistance, which develops after exposure to chemotherapy agents. Small membrane-bound vesicles, known as extracellular vesicles (EVs), are crucial in intercellular signaling as they transport bioactive molecules that can modify the characteristics and functions of recipient cells. Recent research highlights EVs as pivotal players in fostering drug resistance. This review focuses on the intercellular transfer of MDR from donor cells to susceptible recipient cells through specific cargo in EVs, such as ATP-binding cassette (ABC) transporter proteins, nucleic acids, and other regulatory factors. Additionally, the features of intercellular communication mediated by EVs are also discussed. Gaining insight into these mechanisms is essential for developing strategies to counteract resistance and improve the effectiveness of cancer treatments.
Keywords
INTRODUCTION
Extracellular vesicles (EVs) are a recently defined collective term that refers to various types of nano-sized, lipid bilayer-enclosed vesicles[1]. These nanovesicles, once regarded as “cellular waste products”, are now recognized as key regulators of various biological functions and intercellular communication[2]. EVs facilitate cellular information exchange by carrying a diverse array of soluble and insoluble signaling molecules, structural proteins, nucleic acids, and lipids. The molecular cargo and biological functions of EVs are primarily determined by their originating cells[3]. In addition to cell-to-cell contact, EVs can travel through body fluids, delivering their contents to distant target cells, thereby mediating systemic cellular communication in vivo[3-5].
Despite the numerous challenges in the isolation and characterization of EVs, it is increasingly evident that EVs containing functional proteins, nucleic acids, and other bioactive molecules can transfer to recipient cells and modulate their behavior. Recent studies have identified a functional role for EVs in communication between cancer and immune cells. These properties make EVs a promising tool for cancer immunotherapy and diagnostics. EVs are implicated in several aspects of tumor progression, including altering the tumor microenvironment (TME), promoting tumor growth, and facilitating angiogenesis and metastasis[6].
Multidrug resistance (MDR) remains a significant challenge in cancer treatment. Cancer cells can evade the cytotoxic effects of chemotherapeutic agents through intrinsic or acquired resistance mechanisms. Emerging evidence indicates that EVs play a crucial role in developing drug resistance. These vesicles can disseminate resistance to chemotherapy, radiotherapy, targeted therapy, and immunotherapy by transferring specific proteins, nucleic acids, survival signaling molecules, and genetic material to cancer cells[7]. Moreover, EVs contribute to therapy resistance by influencing the interactions between cancer cells and non-cancer cells within the TME[8]. For instance, tumor-derived exosomes can reprogram cancer-associated fibroblasts (CAFs) to create an acidic TME, enhancing resistance to platinum-based drugs in non-small cell lung cancer (NSCLC)[9]. Recently, EVs have emerged as ideal candidates for drug development and delivery due to their stability, biocompatibility, and targeting capabilities[10]. Engineered and synthetic vesicles hold potential as drug delivery vehicles to overcome cancer drug resistance and boost therapeutic efficacy. Understanding the role and mechanisms of EVs in MDR development can help enhance chemotherapy effectiveness and maximize the therapeutic benefits of EVs in cancer treatment. This review briefly summarizes the biogenesis of EVs and highlights key advances in intercellular communication mediated by EVs. We emphasize the “non-genetic” transfer of MDR via specific mediators in EVs, such as ATP-binding cassette (ABC) transporter proteins, nucleic acids, and other regulatory factors.
EVS
EVs are a heterogeneous population that includes exosomes, microvesicles, and apoptotic bodies, which are released by various cell types. These membranous vesicles are generally distinguished by their size and biogenesis[11]. Exosomes, which range from 30 to 150 nm in diameter, are produced through the classical endosome-multivesicular body (MVB) pathway, followed by fusion with the plasma membrane[12]. Microvesicles, ranging from 150 to 1,000 nm in diameter, are shed directly from the plasma membrane. Apoptotic vesicles, even larger vesicles with diameters ranging from 500 to 2,000 nm, form during programmed cell death and are characterized by their substantial amounts of RNA, distinguishing them from other microvesicles[13-15]. However, recent studies have also identified smaller apoptotic exosomes (under 500 nm) that differ in size, content, properties, and function from traditional apoptotic vesicles[16-18]. New EV subtypes have also been discovered, such as oncosomes, which are even larger (1 to 10 μm) and are exclusively shed from the plasma membrane of cancer cells[6]. EV subtypes often share similarities; for example, some microvesicles are less than 150 nm in diameter. Most EVs reported in published studies are actually a mixture of vesicles, primarily because the focus has often been on their potential biological roles rather than their origins. Despite recent advances in understanding EVs, it remains challenging to isolate specific EV classes due to technical limitations in purification and isolation. Furthermore, assigning EVs to particular biogenesis pathways is complicated, as there are no widely recognized specific markers for EV subtypes. The International Society for Extracellular Vesicles (ISEV) has urged authors to use more precise terms to define EVs according to their physical characteristics, biochemical composition, and cell of origin[13].
EVs contain a variety of biologically active molecules, including proteins, nucleic acids, and lipids. The proteins enclosed in EVs include extracellular matrix (ECM) components, membrane proteins, and nuclear proteins. Several tetra-transmembrane proteins (e.g., CD9, CD37, CD81, CD82, and CD63) are particularly abundant in exosomes and microvesicles[19-21]. CD63 and the cytosolic protein heat shock 70kDa protein (HSP70) are frequently used as exosome marker proteins[22,23]. Cytosolic metabolites and nucleic acids (e.g., DNA, noncoding RNA, and mRNA) are also found in exosomes[24,25]. On the outer surface, exosomes feature glycan crowns attached to lipids and proteins, with underlying phospholipids, cholesterol, ceramides, glycerides, and sphingolipids playing key roles in EV biogenesis, uptake, and their functional effects on recipient cells[26-28]. The cargo composition and abundance of EVs vary depending on the type of donor cells, metabolic regulation, and disease states[29]. The process of EV cargo selection is precisely and dynamically regulated. Cargo-driven biogenesis largely contributes to EV heterogeneity, and in some cases, specific cargoes unique to certain cell types can serve as biomarkers for tissue or disease diagnosis[29]. The endosomal sorting complex required for transport (ESCRT) machinery, which is crucial for membrane shaping and scission, is a major regulator of exosome biogenesis and a key determinant of their functional properties[30]. The biogenesis of microvesicles involves multiple molecular rearrangements in the plasma membrane, including those involving lipids, cytoskeletal elements, and their regulators[30]. For instance, depleting membrane cholesterol pharmacologically impairs microvesicle shedding in activated neutrophils[31]. The “Warburg effect”, a hallmark of cancer metabolism, is associated with the biogenesis of oncosomes[32]. In the release process of EVs, Rab family proteins and soluble N‐ethylmaleimide‐sensitive fusion attachment protein receptors (SNAREs) are important regulators[33,34]. Additionally, factors such as low pH, hypoxia, and increased external pressure can promote EV release[8].
Once in the extracellular environment, EVs can enter recipient cells by endocytosis or direct fusion with the target cell membrane[35]. The recipient cells determine the route of EV entry and their subsequent fate[36]. The specific composition of EVs also affects their fate. EVs deliver their contents to elicit phenotypic and functional changes in recipient cells through three main mechanisms: (1) Direct activation of target cells via interactions between surface ligands on the EVs and receptors on the target cells; (2) Transfer of activated receptors; and (3) Genetic reprogramming through the delivery and translation of mRNAs or post-translational modifications[37].
INTERCELLULAR COMMUNICATION OF EVS
EVs are secreted by a diverse range of cell types, including tumor cells, neurons, epithelial cells, and various immune cells such as dendritic cells, T cells, B cells, and mast cells[38]. Initially identified as “cellular dust”, EVs were once believed to serve merely as a means for cells to eliminate unwanted components. However, accumulating research has revealed that EVs are actively involved in transferring information between cells, significantly impacting cellular functions and facilitating intercellular communication.
Intercellular communication via EVs among tumor cells
Growing evidence shows the intercellular transfer of EVs between tumor cells. For example, glioma cells that secrete EVs containing EGFRvIII can fuse with the plasma membranes of other glioma cells lacking EGFRvIII, thereby transferring oncogenic activity[39]. The transfer of EGFR-rich EVs also occurs from highly to poorly metastatic nasopharyngeal carcinoma cells[40]. This transfer of oncogenic signaling components from one cell to another underscores the important role of EVs in cancer. Recent evidence indicates that mRNA and miRNA transferred by EVs from cancer cells can influence tumorigenesis, drug resistance, and tumor progression[41,42]. For example, exosomes from cisplatin (DDP)-resistant cancer cells have been shown to transfer miR-100-5p to recipient lung cancer cells, altering their resistance to DDP[43]. Exosomes released from pancreatic ductal adenocarcinoma (PDAC) cells with long-term exposure to gemcitabine delivered miR-155 to other PDAC cells, inducing chemoresistance[44]. Additionally, exosomes from gemcitabine-resistant A549 cells carrying miR-222-3p have been found to enhance proliferation, gemcitabine resistance, migration, and invasion in sensitive parental cells[45].
Intercellular communication via EVs between non-tumor cells
Intercellular transfers of EVs in non-tumor cells were also reported. For instance, exosomes released by CD4+ T cells can suppress the responses of CD8+ cytotoxic T lymphocytes and thereby inhibit antitumor immunity[46]. Ying et al. demonstrated that exosomes containing miRNA secreted by M1 macrophages can be transferred to adipocytes, causing glucose intolerance and insulin resistance[47]. The transfer of multiple molecules, such as miRNAs, gangliosides, and proteins, by EVs has been found to affect neurodegeneration[48]. Morel et al. characterized a new exosome-mediated transfer of miRNA from neurons to astrocytes[49]. Intercellular communication by EVs between various types of cardiac cells has been widely reported[50]. EVs originating from leukocytes, erythrocytes, smooth muscle cells, and endothelial cells can affect processes such as inflammation, thrombosis, neoangiogenesis, cell survival, and endothelial homeostasis, thus playing a role in the initiation and progression of atherosclerosis[51,52].
Intercellular communication via EVs between tumor cells and non-tumor cells
Intercellular communication of EVs also occurs between cells of different origins. For instance, EVs are transferred between immune cells and tumor cells. Exosomes from hypoxic human epithelial ovarian cancer cells are transferred to macrophages, resulting in the differentiation of macrophages towards an anti-inflammatory M2 phenotype[53]. Colorectal cancer-derived exosomes containing miR-934 promote M2 macrophage polarization and induce pre-metastatic niche formation[54]. Gastric cancer-derived exosomes can be internalized by intrahepatic macrophages, causing M2-like polarization that accelerates liver metastasis of gastric cancer[55]. Accumulating evidence demonstrates that tumor-derived exosomes can transfer to dendritic cells and interfere with their maturation, differentiation, and function[56]. Zhang et al. showed that tumor cell-derived exosomes containing circUHRF1 induce exhaustion in natural killer cells and drive resistance against anti-PD1 immunotherapy[57].
Communication between cancer cells and CAFs mediated by EVs has also been reported. Malignant mesothelioma-derived exosomes are suggested to facilitate the migratory capacity of CAFs[58]. Zhao et al. revealed that CAF-derived exosomes transport essential nutrients to cancer cells and modulate cancer cell metabolism[59]. Tumor-derived EVs interact with endothelial cells to exhibit proangiogenic activities by delivering activated EGFR[60].
INTERCELLULAR TRANSFER OF MDR BY EVS
MDR is characterized by the cross-resistance of cancer cells to a range of chemotherapeutic drugs that have distinct structures and mechanisms of action[61]. This resistance significantly contributes to the failure of chemotherapy and is commonly associated with the recurrence of tumors. MDR can be categorized into two types: intrinsic resistance and acquired resistance. The former refers to pre-existence before the use of chemotherapeutic drugs, and the latter arises by multiple mechanisms after exposure to chemotherapeutics. Several potential mechanisms underlying MDR have been identified in cancer cells, including reduced drug uptake, increased drug efflux by the ABC transporter family, regulation by cancer stem cells, altered DNA damage response and repair, induction of hypoxia, altered drug targets and sequestration of anticancer drugs in intracellular organelles[61-67]. Among these mechanisms, the high expression of the ABC transporter family is the predominant molecular mechanism underlying MDR in cancer cells. Recent studies have revealed a novel mechanism responsible for MDR, in which EVs directly transport various proteins, including ABC transporters and proteins related to tumor survival and DNA repair, to recipient cells [Table 1]. Additionally, EVs can transport RNAs, including miRNAs and long noncoding RNAs (lncRNAs), from donor cells to recipient cells, which can lead to the development of drug resistance[68] [Table 1]. Furthermore, the intercellular transfer of EVs can reshape the TME, creating conditions that favor therapy resistance[69,70].
Diverse cargo types in EV-mediated transfer of MDR
Cargo type | Molecules | Drug resistance | Model | Refs. |
Drug efflux pumps | ABCB1 | Colchicine | Human neuroblastoma cell line BE(2)-C, and human breast adenocarcinoma cell line MCF-7 | [78] |
Carboplatine, taxol | Primary stromal cells and human ovarian cancer cell line OVCAR3 | [79] | ||
Daunorubicin | Human acute lymphoblastic leukemia cell line CCRF-CEM | [80] | ||
Docetaxel | Human prostate cancer cell lines 22Rv1, DU145 and LNCap | [81] | ||
NA | Human acute lymphoblastic leukaemia cell line CCRF-CEM, and human breast adenocarcinoma cell line MCF-7 | [82] | ||
Docetaxel | Human breast adenocarcinoma cell line MCF-7 | [83] | ||
NA | Human breast adenocarcinoma cell line MCF-7 | [84] | ||
NA | Human bladder cancer cell line BIU-87 | [86] | ||
Doxorubicin | Human bladder cancer cell line BIU-87 | [87] | ||
Adriamycin, paclitaxel | Human ovarian cancer cell line A2780 | [88] | ||
Doxorubicin | Human osteosarcoma cell line MG-63 | [89] | ||
Doxorubicin | Human oral epidermoid carcinoma cell line KB, and human colon carcinoma cell line S1 | [90] | ||
ABCC1 | NA | Human acute lymphoblastic leukaemia cell CCRF-CEM, and human breast adenocarcinoma cell line MCF7 | [94] | |
Daunorubicin | Human acute promyelocytic leukemia cell line HL60 | [95] | ||
Nucleic acids | miR-27a and miR-326 | NA | Human acute lymphoblastic leukemia cell line CCRF-CEM, and human breast adenocarcinoma cell line MCF-7 | [106] |
miR-100, miR-222, and miR-30a | NA | Human breast adenocarcinoma cell line MCF-7 | [7] | |
miR‐21‐5p | DDP | Human ovarian cancer cell line SKOV3 | [107] | |
miR-301b-3p | DDP/VCR | Human gastric cancer cells | [108] | |
miR-221/222 | Tamoxifen | Human breast adenocarcinoma cell line MCF-7 | [109] | |
Other regulators | TrpC5 | Adriamycin | Human breast adenocarcinoma cell line MCF-7 | [113] |
EGFR | Osimertinib | Human NSCLC cell lines H460, A549, H1299, H1975, and PC9 | [114] | |
PDGFRβ | PLX4720 | Human melanoma cell line LM-MEL-64 | [115] | |
HGF | Sorafenib | Human hepatocellular carcinoma cell line SMMC-7721, MHCC-97H, and MHCC-97 L | [117] | |
Survivin | Paclitaxel | Human breast cancer cell line MDA-MB-231 | [119] |
Transfer of ABC transporter proteins by EVs
ABC transporters are a family of membrane proteins that utilize ATP hydrolysis to transport a diverse array of substrates, including ions and macromolecules, across extra- and intercellular membranes. The human ABC transporter family includes 48 members, classified into seven subfamilies (A to G) based on sequence homology, domain organization, and phylogenetic analysis[71]. A canonical ABC transporter consists of two highly variable transmembrane domains (TMDs) embedded in the membrane bilayer and two highly conserved nucleotide-binding domains (NBDs) located in the cytoplasm. The TMDs provide the specificity for the substrate and the NBDs transfer energy to transport the substrate across the membrane[71]. Based on their function, ABC transporters are divided into three classes: exporters, importers, and non-transporters. Although most ABC transporters are exporters, ABCA4 and ABCD4 function as importers[72,73]. The ABCE and ABCF families do not function as transporters but act as regulators of mRNA translation[74]. Over the past decade, at least 13 ABC transporters have been implicated in the development of MDR in vitro. The high expression of ABC transporters, particularly ABCB1, ABCC1, and breast cancer resistance protein (BCRP), is a widely recognized molecular mechanism that contributes to MDR in cancer cells. More than 50% of cancers exhibiting an MDR phenotype have been found to express these ABC transporters constitutively or inducibly, enabling them to expel chemotherapeutic drugs and reduce intracellular drug concentrations[75].
ABCB1
ABCB1, also known as P-glycoprotein (P-gp), is the first and most extensively studied member of the ABC transporter family. It is closely associated with the MDR phenotype by actively exporting a broad spectrum of chemotherapeutic agents from cancer cells. In mammals, particularly in humans, ABCB1 is prominently expressed in specific tissues such as the intestine, liver, kidney, and brain, where it plays a key role in drug transport and detoxification processes[76]. ABCB1 consists of two homologous NBDs and two homologous TMDs. The NBDs create a large pocket essential for ATP binding and hydrolysis, which is critical for ATPase activity and energy generation. Meanwhile, the TMDs form a flexible channel with 12 transmembrane helices responsible for substrate recognition and transportation[76]. Clinical studies have demonstrated that ABCB1 is overexpressed in various malignant tumor cells and is associated with chemotherapy efficacy and cancer progression[77]. The increased expression of ABCB1 in cancer cells enhances the efflux of various structurally and mechanistically different anticancer drugs, such as paclitaxel, docetaxel, vincristine, doxorubicin, imatinib, and nilotinib, leading to decreased intracellular drug levels and subsequent chemoresistance.
The intercellular transfer of ABCB1 via EVs has been supported by extensive experimental evidence. Levchenko et al. first reported in 2005 that functional ABCB1 can transfer from drug-resistant to drug-sensitive cancer cells both in vitro and in vivo, resulting in increased drug resistance without the expression of the ABCB1 gene[78]. This transfer was mediated by relatively large EVs (> 0.8 μm in diameter) or required cell-to-cell contact, but not by small EVs (below 0.2-μm in diameter)[78]. This study also demonstrated that the functional transfer of ABCB1 occurred across different tumor cell types, suggesting a broad mechanism for the dissemination of MDR[78]. Subsequent studies have further supported the intercellular transfer of ABCB1. For instance, Rafii et al. found that ovarian cancer cells gain chemoresistance through the transfer of functional ABCB1 proteins from an original type of stromal cells[79]. In acute lymphoblastic leukemia cells, ABCB1 proteins carried by EVs from drug-resistant cells were found to transfer into drug-sensitive recipient cells, leading to an MDR phenotype within as early as 2 h[80]. The rapid transfer of ABCB1 by EVs and the swift acquisition of drug resistance in recipient cells within two hours suggest that this process likely involves the direct transfer of exogenous ABCB1 protein from donor cells, rather than the upregulation of ABCB1 gene expression in recipient cells. Corcoran et al. demonstrated that exosomes derived from docetaxel-resistant prostate cancer cells notably enhanced docetaxel resistance in previously sensitive recipient cells, partly attributed to the transfer of ABCB1[81]. Similarly, Jaiswal et al. observed a stable transfer of ABCB1 mediated by microparticles (MPs) in both in vitro and in vivo settings[82]. Comparable transfer of drug resistance and ABCB1 protein by EVs have been reported in breast cancer, bladder cancer, and ovarian cancer cells[83-88]. Additionally, drug-resistant osteosarcoma cells have been shown to transfer exosomes containing both ABCB1 protein and mRNA, thereby conferring doxorubicin resistance to sensitive cells[89]. Our recent study indicates that in the presence of chemotherapeutic drugs, the intercellular transfer of ABCB1 is enhanced through the stimulated secretion and recycling of ABCB1-enriched EVs, suggesting a higher efficiency of this transfer under selective pressure from chemotherapeutic agents[90].
Jaiswal et al. found that the MDR phenotype acquired by recipient cells remained stable for at least 5 days in vitro, even in the absence of selecting pressure[91]. In an MCF-7 tumor xenograft model, the MDR phenotype can be rapidly acquired by drug-sensitive cancer cells within 24 h and can persist for at least 2 weeks in vivo[82]. The MDR phenotype transferred by EVs protected sensitive tumor cells for up to 4 months, facilitating the development of intrinsic ABCB1-mediated resistance[78]. However, the transfer of ABCB1 protein via EVs alone does not fully account for the long-term persistence of this MDR phenotype, especially considering that the half-life of ABCB1 is approximately 14 to 17 h. One plausible explanation is that other cargo, such as miRNAs, is delivered to recipient cells alongside ABCB1 by EVs.
ABCC1
ABCC1, also known as multidrug resistance-associated protein 1 (MRP1), plays a significant role in the recognition and efflux of both hydrophobic and hydrophilic antineoplastic agents, resulting in decreased drug accumulation within tumor cells and contributing to the development of MDR. Elevated levels of ABCC1 are strongly associated with poor prognosis and decreased survival across multiple cancer types[92,93].
Emerging evidence suggests that ABCC1 is also involved in the transfer of MDR between cells via EVs. For example, Lu et al. reported the intercellular transfer of functional ABCC1 via MPs[94]. Lymphoblastic leukemia cells that overexpress ABCC1 spontaneously shed MPs containing ABCC1, which can then transfer to drug-sensitive cells within 12 h of co-culture[94]. Another study revealed that chemoresistance can be transmitted from drug-resistant leukemia cells to their parental counterparts via EVs, likely due to the direct transfer of ABCC1[95].
ABCG2
ABCG2, also known as BCRP, is a “half-transporter” featuring a single NBD and a single TMD[96]. Unlike other ABC transporters, ABCG2 functions as a homodimer, comprising two identical subunits. The dimerization of ABCG2 is essential for its transport activity, as the NBDs must dimerize to form a functional structure necessary for ATP binding and hydrolysis[97]. ABCG2 is highly expressed in the placenta, blood-brain barrier, liver, and intestine, and is notably prevalent in various stem cells, including hematopoietic stem cells. Its expression is associated with the “side population” phenotype, which is associated with stemness and drug efflux capability[98]. ABCG2 is also a well-recognized transporter involved in MDR across various tumor types. It has been reported to transport several anticancer drugs, including mitoxantrone, topotecan, and irinotecan. The ability of ABCG2 to expel a wide range of drugs from cancer cells enables these cells to evade the cytotoxic effects of chemotherapy. Elevated ABCG2 expression has been implicated in cancer progression and poor clinical outcomes[99].
Recent studies have emphasized the role of ABCG2 in the function and composition of EVs. ABCG2 is abundantly expressed on the membrane of EVs secreted by cancer cells. These EVs can encapsulate and export chemotherapeutic drugs out of the cell, reducing intracellular drug concentrations and contributing to enhanced drug resistance. Ifergan et al. suggest that mitoxantrone resistance may result from its entrapment within the vesicular lumen of EVs mediated by overexpressed ABCG2 on the EV membrane[100]. They also suggest that the levels of MDR mediated by ABCG2-rich EVs can be assessed by measuring the ABCG2-dependent intravesicular concentration of riboflavin in EVs[101]. Additionally, ABCG2-enriched EVs can concentrate and sequester various antitumor drugs, increasing the resistance of EVs-forming breast cancer cells to topotecan, imidazoacridinones, and methotrexate[102]. This proposed a novel MDR mechanism where ABC transporters are specifically sorted to the EV membrane, actively sequestering anticancer drugs inside EVs and leading to significant chemoresistance[102]. Moreover, the PI3K-Akt signaling pathway can inhibit the capacity of EVs to sequester anticancer drugs by gradually retracting ABCG2 from the EV membrane into the cytoplasm[103]. As a crucial regulator of ABCG2 localization, suppression of the PI3K-Akt signaling pathway decreases the sorting of ABCG2 to EVs, eventually reducing EV production and reversing MDR. Further studies suggest that the size and number of EVs gradually decrease due to the retention of intracellular ABCG2[103]. These findings have provided a wealth of information on the role of ABCG2 in the biogenesis of EVs. However, to date, there is no direct evidence suggesting the spread of MDR through the intercellular transfer of ABCG2-containing EVs, which requires further investigation.
Transfer of nucleic acids by EVs
EVs derived from cancer cells carry various types of genetic material, including mRNA, lncRNAs, ribosomal RNAs (rRNAs), and small nuclear RNAs (snRNAs), all of which are functionally active[104]. Recent research suggests that EVs play a role in the dissemination of MDR through intercellular nucleic acid exchange, particularly via miRNAs[105]. miRNAs are single-stranded, noncoding RNA that regulate specific mRNA targets and are implicated in the development of chemoresistance by influencing multiple genes. The aberrant expression of multiple miRNAs has been implicated in resistance to various anticancer drugs such as topotecan, doxorubicin, methotrexate, docetaxel, and DDP. Earlier studies emphasized the crucial role of miRNAs within EVs in the dissemination of MDR. For example, Jaiswal et al. provided evidence that transporter transcripts and regulatory nucleic acids (miRNAs) can be transferred via EVs isolated from ABCB1-overexpressing multidrug-resistant leukemia and breast cancer cells. These EVs can alter the transcriptional environment of recipient cells, thereby reflecting the donor MDR phenotype[106]. Recent studies have pinpointed specific miRNAs within EVs that contribute to MDR dissemination. Chen et al. demonstrated that EVs from drug-resistant breast cancer cells convey chemoresistance in an RNA-dependent manner, with microRNAs such as miR-100, miR-222, and miR-30a being transferred to sensitive recipient cells. Further transfection experiments with miRNA mimics indicated that miR-222 mimics could regulate drug resistance by targeting PTEN, with miR-222-rich EVs altering PTEN expression in drug-sensitive breast cancer cells[7]. Similarly, exosomal miR-21-5p has been shown to influence cancer cell chemosensitivity by targeting pyruvate dehydrogenase E1 subunit α 1 (PDHA1). In DDP-resistant SKOV3 cells and tumor tissues, miR-21-5p expression was upregulated, while PDHA1 levels were significantly decreased. Exosomes from DDP-resistant ovarian cancer cells increased the resistance of sensitive cells to DDP and promoted cell viability by targeting PDHA1 with exosomal miR-21-5p[107]. Zhu et al. reported that EVs carrying miR-301b-3p from mesenchymal stem cells (MSCs) induced MDR in gastric cancer cells by suppressing thioredoxin-interacting protein (TXNIP)[108]. Inhibition of miR-301b-3p or upregulation of TXNIP increased the chemosensitivity of DDP/VCR-resistant gastric cancer cells and reversed malignant behaviors. Another study suggests that EVs carrying miR-221/222 transmit tamoxifen resistance to drug-sensitive breast cancer cells[109]. Exosomes from tamoxifen-resistant breast cancer cells can enter tamoxifen-sensitive cells and release miR-221/222, leading to enhanced resistance by reducing the expression of p27 and ERα, targets of miR-221/222[109].
EVs are emerging as effective and promising vehicles for drug delivery due to their natural properties of low immunogenicity and high biocompatibility[110]. EVs can protect miRNAs from degradation by RNases, unlike naked miRNAs, opening new avenues for overcoming MDR. For instance, miR-9 regulates the expression of the drug efflux transporter ABCB1. Munoz et al. suggested that microvesicles are the primary carriers of anti-miR-9 from MSCs to glioblastoma multiforme cells, reversing chemoresistance by downregulating ABCB1[111]. Similarly, paclitaxel-sensitive NPC cells have been reported to deliver miR-183-5p to paclitaxel-resistant NPC cells via EVs, thereby suppressing paclitaxel resistance by negatively regulating ABCB1[112].
Transfer of other regulators by EVs
In addition to ABC transporters, other proteins transported via EVs may also significantly contribute to the development of MDR. Ma et al. found that transient receptor potential channel 5 (TrpC5) can be delivered to sensitive cancer cells via EVs derived from drug-resistant breast cancer cells. This transfer enables the recipient cells to acquire the Ca2+-permeable channel, which in turn stimulates P-gp expression through a Ca2+- and NFATc3-mediated mechanism, ultimately conferring chemoresistance to the previously sensitive cells[113]. A recent study suggested that exosomes can transfer wild-type EGFR, which, upon internalization by EGFR-mutated cancer cells, activates downstream PI3K/AKT and MAPK signaling pathways, thereby conferring osimertinib resistance to EGFR-mutated sensitive cancer cells both in vitro and in vivo[114]. Platelet-derived growth factor receptor-beta (PDGFRβ), a critical factor in resistance, is enriched in EVs released by melanoma cells that are resistant to the BRAF inhibitor PLX4720. Upon transfer to recipient melanoma cells, PDGFRβ triggers a dose-dependent activation of the PI3K/AKT signaling pathway, enabling these cells to circumvent BRAF inhibition[115]. Ji et al. found that exosomes originating from MSCs induce resistance to 5-fluorouracil in gastric cancer cells, with elevated expression of multiple drug-resistance-associated proteins, including ABCB1, ABCC1, and LRP1[116]. Interestingly, MSC exosome-delivered proteins, rather than ABCB1 alone, played a dominant role in acquired resistance[116].
EVs also transport molecules that promote cell survival, including prosurvival factors and proteins involved in apoptosis regulation. By improving the viability of recipient cells and decreasing their susceptibility to apoptosis, these components contribute to the development of MDR. For instance, in hepatocellular carcinoma (HCC) cell lines, EVs carrying hepatocyte growth factor (HGF) have been shown to induce resistance to Sorafenib both in vitro and in vivo. This resistance is mediated by the activation of the HGF/c-MET/PI3K/AKT signaling pathway, which is crucial for cell survival and drug resistance[117]. Additionally, EVs from apoptosis-resistant acute myeloid leukemia (AML) blasts contain proteins that regulate apoptosis and impart a drug-resistant phenotype to primary AML cells that are initially sensitive to apoptosis[118]. Survivin, a prosurvival protein and a member of the inhibitors of apoptosis (IAP) family, plays a vital role in preventing cell death and regulating mitosis. Kreger et al. reported that treatment of highly aggressive MDA-MB-231 breast cancer cells with paclitaxel stimulates the release of EVs rich in survivin. These survivin-enriched EVs significantly enhance the survival of serum-starved and PTX-treated fibroblasts and SKBR3 breast cancer cells[119].
Beyond the direct transfer of bioactive molecules, EVs also reshaped the TME, facilitating the transmission of MDR. Cell-to-cell communication within the TME occurs through direct contact or the exchange of bioactive molecules via EVs. EVs are recognized as key regulators of tumor dynamics. Lopes-Rodrigues
STRATEGIES TO OVERCOME EV-MEDIATED DRUG RESISTANCE
Given the pivotal role of EVs in MDR, it is crucial to develop strategies to counteract this form of drug resistance. One direct approach involves inhibiting the production and release of EVs from drug-resistant cells. Various pharmacological agents have been identified that can interfere with EV formation and release, thereby enhancing the efficacy of anticancer treatments. GW4869, a known inhibitor of neutral sphingomyelinase, is commonly used to impede EV production and diminish EV release. Research by Hekmatirad et al. showed that GW4869 effectively prevents the efflux of PEGylated liposomal doxorubicin (PLD) and increases U937 cell sensitivity to PLD’s cytotoxic effects[124]. Similarly, blocking EV release from CAFs with GW4869 reduces the transfer of chemoresistance and decreases the survival of pancreatic cancer cells[123]. Moreover, extracellular signal-regulated kinase (ERK) has been linked to EV release. Using the MEK inhibitor U0126 to block ERK activation sensitizes gemcitabine-resistant pancreatic cells to gemcitabine, both in vitro and in vivo[125]. Federici et al. found that Lansoprazole, a proton pump inhibitor, reduces overall EV release from tumor cells, thereby enhancing sensitivity to DDP[126]. Additionally, Rab GTPases, which are critical for EV trafficking and release, can be targeted. For example, siRNA-mediated inhibition of Rab27a reduces EV release and significantly decreases miR-155-induced gemcitabine resistance in pancreatic cancer cells[44].
Another approach involves preventing the uptake of EVs by recipient cells. Disrupting the interaction between EVs and their target cells can limit the transfer of MDR-associated factors. Heparin, a glycosaminoglycan, can inhibit EV binding to cell surface proteins, thereby reducing EV uptake. Studies have demonstrated that heparin treatment significantly decreases the transfer of EVs containing EGFRvIII mRNA between tumor cells[127]. EVs are frequently internalized through macropinocytosis, and inhibitors of this process, such as 5-(N-ethyl-N-isopropyl) amiloride (EIPA), can block EV uptake and restore EGFR-TKI sensitivity in PC-9 cells[128].
Despite the promising potential of these strategies to overcome EV-mediated MDR, several challenges must be addressed to ensure their clinical effectiveness. The heterogeneity of EVs in size, content, and function complicates the development of universal targeting strategies. Additionally, targeting EVs may affect normal physiological processes, as EVs are produced by both cancerous and healthy cells and play crucial roles in immune modulation. Inhibiting EVs might inadvertently disrupt beneficial immune responses, potentially leading to tumor progression or immune-related side effects. Furthermore, designing effective therapies to block EV-mediated MDR presents technical difficulties due to the need for precise molecular targeting. Future research should aim to enhance our understanding of EV biology, refine targeting methods, and address potential resistance mechanisms.
CONCLUSION
Tumor MDR represents a significant challenge in cancer therapy, often arising from complex genetic alterations induced by drug exposure. However, recent findings underscore a novel non-genetic mechanism in which EVs mediate the transfer of drug resistance between cells. Specifically, EVs from drug-resistant tumor cells can horizontally transfer various components, such as drug-efflux pumps (e.g., ABCB1, ABCC1), miRNAs (e.g., miR-21-5p, miR-222), and regulatory proteins (e.g., TrpC5, EGFR), to drug-sensitive cells, thereby spreading a drug-resistant phenotype. Furthermore, EV-mediated intercellular communication within the TME plays a crucial role in MDR propagation. These insights into non-genetic mechanisms of MDR not only provide opportunities for developing novel therapeutic strategies to overcome MDR but also hold promise for identifying biomarkers of drug resistance. Despite remarkable progress, the intercellular transfer of MDR via EVs remains poorly understood. Key aspects requiring further investigation include understanding how specific molecules are selected for inclusion in EVs released by donor cells, the regulatory mechanisms that control EV production, and the processes governing EV uptake and recycling in recipient cells. Additionally, clinical studies are essential to elucidate the clinical relevance of this non-genetic mode of MDR transmission, its impact on patient response to chemotherapy, and overall clinical outcome. Addressing these gaps will be critical for advancing our understanding and improving treatment outcomes in cancer therapy.
DECLARATIONS
Authors’ contributions
Drafted the manuscript and organized the figures and tables: Yang A, Sun H
Supervised the conceptualization of the review and revised the manuscript: Wang X
Availability of data and materials
Not applicable.
Financial support and sponsorship
This work was supported by the Fundamental Research Funds for Hainan University [KYQD(ZR)23146, China].
Conflicts of interest
All authors declared that there are no conflicts of interest.
Ethical approval and consent to participate
Not applicable.
Consent for publication
Not applicable.
Copyright
© The Author(s) 2024.
REFERENCES
1. György B, Szabó TG, Pásztói M, et al. Membrane vesicles, current state-of-the-art: emerging role of extracellular vesicles. Cell Mol Life Sci 2011;68:2667-88.
2. Gurung S, Perocheau D, Touramanidou L, Baruteau J. The exosome journey: from biogenesis to uptake and intracellular signalling. Cell Commun Signal 2021;19:47.
3. Skog J, Würdinger T, van Rijn S, et al. Glioblastoma microvesicles transport RNA and proteins that promote tumour growth and provide diagnostic biomarkers. Nat Cell Biol 2008;10:1470-6.
4. Peinado H, Alečković M, Lavotshkin S, et al. Melanoma exosomes educate bone marrow progenitor cells toward a pro-metastatic phenotype through MET. Nat Med 2012;18:883-91.
5. Ratajczak J, Miekus K, Kucia M, et al. Embryonic stem cell-derived microvesicles reprogram hematopoietic progenitors: evidence for horizontal transfer of mRNA and protein delivery. Leukemia 2006;20:847-56.
6. Minciacchi VR, Freeman MR, Di Vizio D. Extracellular vesicles in cancer: exosomes, microvesicles and the emerging role of large oncosomes. Semin Cell Dev Biol 2015;40:41-51.
7. Chen WX, Liu XM, Lv MM, et al. Exosomes from drug-resistant breast cancer cells transmit chemoresistance by a horizontal transfer of microRNAs. PLoS One 2014;9:e95240.
8. Yang Q, Xu J, Gu J, et al. Extracellular vesicles in cancer drug resistance: roles, mechanisms, and implications. Adv Sci 2022;9:e2201609.
9. Wang D, Zhao C, Xu F, et al. Cisplatin-resistant NSCLC cells induced by hypoxia transmit resistance to sensitive cells through exosomal PKM2. Theranostics 2021;11:2860-75.
10. Marar C, Starich B, Wirtz D. Extracellular vesicles in immunomodulation and tumor progression. Nat Immunol 2021;22:560-70.
11. Gould SJ, Raposo G. As we wait: coping with an imperfect nomenclature for extracellular vesicles. J Extracell Vesicles 2013;2:20389.
12. Hessvik NP, Llorente A. Current knowledge on exosome biogenesis and release. Cell Mol Life Sci 2018;75:193-208.
13. Théry C, Witwer KW, Aikawa E, et al. Minimal information for studies of extracellular vesicles 2018 (MISEV2018): a position statement of the International Society for Extracellular Vesicles and update of the MISEV2014 guidelines. J Extracell Vesicles 2018;7:1535750.
14. Bayraktar R, Van Roosbroeck K, Calin GA. Cell-to-cell communication: microRNAs as hormones. Mol Oncol 2017;11:1673-86.
15. Battistelli M, Falcieri E. Apoptotic bodies: particular extracellular vesicles involved in intercellular communication. Biology 2020;9:21.
16. Pavlyukov MS, Yu H, Bastola S, et al. Apoptotic cell-derived extracellular vesicles promote malignancy of glioblastoma via intercellular transfer of splicing factors. Cancer Cell 2018;34:119-35.e10.
17. Poon IKH, Parkes MAF, Jiang L, et al. Moving beyond size and phosphatidylserine exposure: evidence for a diversity of apoptotic cell-derived extracellular vesicles in vitro. J Extracell Vesicles 2019;8:1608786.
18. Dieudé M, Bell C, Turgeon J, et al. The 20S proteasome core, active within apoptotic exosome-like vesicles, induces autoantibody production and accelerates rejection. Sci Transl Med 2015;7:318ra200.
19. Escola JM, Kleijmeer MJ, Stoorvogel W, Griffith JM, Yoshie O, Geuze HJ. Selective enrichment of tetraspan proteins on the internal vesicles of multivesicular endosomes and on exosomes secreted by human B-lymphocytes. J Biol Chem 1998;273:20121-7.
20. Tkach M, Kowal J, Théry C. Why the need and how to approach the functional diversity of extracellular vesicles. Philos Trans R Soc Lond B Biol Sci 2018;373:20160479.
21. Andreu Z, Yáñez-Mó M. Tetraspanins in extracellular vesicle formation and function. Front Immunol 2014;5:442.
22. Yanatori I, Richardson DR, Dhekne HS, Toyokuni S, Kishi F. CD63 is regulated by iron via the IRE-IRP system and is important for ferritin secretion by extracellular vesicles. Blood 2021;138:1490-503.
23. Yoshioka Y, Konishi Y, Kosaka N, Katsuda T, Kato T, Ochiya T. Comparative marker analysis of extracellular vesicles in different human cancer types. J Extracell Vesicles 2013;2:20424.
24. Keerthikumar S, Chisanga D, Ariyaratne D, et al. ExoCarta: a web-based compendium of exosomal cargo. J Mol Biol 2016;428:688-92.
25. Pathan M, Fonseka P, Chitti SV, et al. Vesiclepedia 2019: a compendium of RNA, proteins, lipids and metabolites in extracellular vesicles. Nucleic Acids Res 2019;47:D516-9.
26. Skotland T, Sandvig K, Llorente A. Lipids in exosomes: current knowledge and the way forward. Prog Lipid Res 2017;66:30-41.
28. Donoso-Quezada J, Ayala-Mar S, González-Valdez J. The role of lipids in exosome biology and intercellular communication: Function, analytics and applications. Traffic 2021;22:204-20.
29. Dixson AC, Dawson TR, Di Vizio D, Weaver AM. Context-specific regulation of extracellular vesicle biogenesis and cargo selection. Nat Rev Mol Cell Biol 2023;24:454-76.
30. van Niel G, D’Angelo G, Raposo G. Shedding light on the cell biology of extracellular vesicles. Nat Rev Mol Cell Biol 2018;19:213-28.
31. Del Conde I, Shrimpton CN, Thiagarajan P, López JA. Tissue-factor-bearing microvesicles arise from lipid rafts and fuse with activated platelets to initiate coagulation. Blood 2005;106:1604-11.
32. Wilson KF, Erickson JW, Antonyak MA, Cerione RA. Rho GTPases and their roles in cancer metabolism. Trends Mol Med 2013;19:74-82.
33. Milman N, Ginini L, Gil Z. Exosomes and their role in tumorigenesis and anticancer drug resistance. Drug Resist Updat 2019;45:1-12.
34. Colombo M, Raposo G, Théry C. Biogenesis, secretion, and intercellular interactions of exosomes and other extracellular vesicles. Annu Rev Cell Dev Biol 2014;30:255-89.
35. Zhang X, Yuan X, Shi H, Wu L, Qian H, Xu W. Exosomes in cancer: small particle, big player. J Hematol Oncol 2015;8:83.
36. Mulcahy LA, Pink RC, Carter DRF. Routes and mechanisms of extracellular vesicle uptake. J Extracell Vesicles 2014;3:24641.
37. Antebi YE, Nandagopal N, Elowitz MB. An operational view of intercellular signaling pathways. Curr Opin Syst Biol 2017;1:16-24.
38. Fu W, Lei C, Liu S, et al. CAR exosomes derived from effector CAR-T cells have potent antitumour effects and low toxicity. Nat Commun 2019;10:4355.
39. Al-Nedawi K, Meehan B, Micallef J, et al. Intercellular transfer of the oncogenic receptor EGFRvIII by microvesicles derived from tumour cells. Nat Cell Biol 2008;10:619-24.
40. Li F, Zhao X, Sun R, et al. EGFR-rich extracellular vesicles derived from highly metastatic nasopharyngeal carcinoma cells accelerate tumour metastasis through PI3K/AKT pathway-suppressed ROS. J Extracell Vesicles 2020;10:e12003.
41. Le MTN, Hamar P, Guo C, et al. miR-200-containing extracellular vesicles promote breast cancer cell metastasis. J Clin Invest 2014;124:5109-28.
42. Melo SA, Sugimoto H, O’Connell JT, et al. Cancer exosomes perform cell-independent microRNA biogenesis and promote tumorigenesis. Cancer Cell 2014;26:707-21.
43. Qin X, Yu S, Zhou L, et al. Cisplatin-resistant lung cancer cell-derived exosomes increase cisplatin resistance of recipient cells in exosomal miR-100-5p-dependent manner. Int J Nanomedicine 2017;12:3721-33.
44. Mikamori M, Yamada D, Eguchi H, et al. MicroRNA-155 controls exosome synthesis and promotes gemcitabine resistance in pancreatic ductal adenocarcinoma. Sci Rep 2017;7:42339.
45. Wei F, Ma C, Zhou T, et al. Exosomes derived from gemcitabine-resistant cells transfer malignant phenotypic traits via delivery of miRNA-222-3p. Mol Cancer 2017;16:132.
46. Zhang H, Xie Y, Li W, Chibbar R, Xiong S, Xiang J. CD4+ T cell-released exosomes inhibit CD8+ cytotoxic T-lymphocyte responses and antitumor immunity. Cell Mol Immunol 2011;8:23-30.
47. Ying W, Riopel M, Bandyopadhyay G, et al. Adipose tissue macrophage-derived exosomal miRNAs can modulate in vivo and in vitro insulin sensitivity. Cell 2017;171:372-84.e12.
48. Chen JJ, Zhao B, Zhao J, Li S. Potential roles of exosomal microRNAs as diagnostic biomarkers and therapeutic application in Alzheimer’s disease. Neural Plast 2017;2017:7027380.
49. Morel L, Regan M, Higashimori H, et al. Neuronal exosomal miRNA-dependent translational regulation of astroglial glutamate transporter GLT1. J Biol Chem 2013;288:7105-16.
50. Poe AJ, Knowlton AA. Exosomes as agents of change in the cardiovascular system. J Mol Cell Cardiol 2017;111:40-50.
51. Leroyer AS, Isobe H, Lesèche G, et al. Cellular origins and thrombogenic activity of microparticles isolated from human atherosclerotic plaques. J Am Coll Cardiol 2007;49:772-7.
52. Boulanger CM, Loyer X, Rautou PE, Amabile N. Extracellular vesicles in coronary artery disease. Nat Rev Cardiol 2017;14:259-72.
53. Chen X, Ying X, Wang X, Wu X, Zhu Q, Wang X. Exosomes derived from hypoxic epithelial ovarian cancer deliver microRNA-940 to induce macrophage M2 polarization. Oncol Rep 2017;38:522-8.
54. Zhao S, Mi Y, Guan B, et al. Tumor-derived exosomal miR-934 induces macrophage M2 polarization to promote liver metastasis of colorectal cancer. J Hematol Oncol 2020;13:156.
55. Qiu S, Xie L, Lu C, et al. Gastric cancer-derived exosomal miR-519a-3p promotes liver metastasis by inducing intrahepatic M2-like macrophage-mediated angiogenesis. J Exp Clin Cancer Res 2022;41:296.
56. Hosseini R, Asef-Kabiri L, Yousefi H, et al. The roles of tumor-derived exosomes in altered differentiation, maturation and function of dendritic cells. Mol Cancer 2021;20:83.
57. Zhang PF, Gao C, Huang XY, et al. Cancer cell-derived exosomal circUHRF1 induces natural killer cell exhaustion and may cause resistance to anti-PD1 therapy in hepatocellular carcinoma. Mol Cancer 2020;19:110.
58. Greening DW, Ji H, Chen M, et al. Secreted primary human malignant mesothelioma exosome signature reflects oncogenic cargo. Sci Rep 2016;6:32643.
59. Zhao H, Yang L, Baddour J, et al. Tumor microenvironment derived exosomes pleiotropically modulate cancer cell metabolism. Elife 2016;5:e10250.
60. Al-Nedawi K, Meehan B, Kerbel RS, Allison AC, Rak J. Endothelial expression of autocrine VEGF upon the uptake of tumor-derived microvesicles containing oncogenic EGFR. Proc Natl Acad Sci U S A 2009;106:3794-9.
61. Wu Q, Yang Z, Nie Y, Shi Y, Fan D. Multi-drug resistance in cancer chemotherapeutics: mechanisms and lab approaches. Cancer Lett 2014;347:159-66.
62. Mansoori B, Mohammadi A, Davudian S, Shirjang S, Baradaran B. The different mechanisms of cancer drug resistance: a brief review. Adv Pharm Bull 2017;7:339-48.
63. Nathanson DA, Gini B, Mottahedeh J, et al. Targeted therapy resistance mediated by dynamic regulation of extrachromosomal mutant EGFR DNA. Science 2014;343:72-6.
64. Kreso A, O’Brien CA, van Galen P, et al. Variable clonal repopulation dynamics influence chemotherapy response in colorectal cancer. Science 2013;339:543-8.
65. Arora VK, Schenkein E, Murali R, et al. Glucocorticoid receptor confers resistance to antiandrogens by bypassing androgen receptor blockade. Cell 2013;155:1309-22.
66. Gatenby RA, Gillies RJ, Brown JS. Evolutionary dynamics of cancer prevention. Nat Rev Cancer 2010;10:526-7.
67. Gong J, Luk F, Jaiswal R, George AM, Grau GE, Bebawy M. Microparticle drug sequestration provides a parallel pathway in the acquisition of cancer drug resistance. Eur J Pharmacol 2013;721:116-25.
68. Bach DH, Hong JY, Park HJ, Lee SK. The role of exosomes and miRNAs in drug-resistance of cancer cells. Int J Cancer 2017;141:220-30.
69. Sharma A. Chemoresistance in cancer cells: exosomes as potential regulators of therapeutic tumor heterogeneity. Nanomedicine 2017;12:2137-48.
70. Camussi G, Deregibus MC, Bruno S, Grange C, Fonsato V, Tetta C. Exosome/microvesicle-mediated epigenetic reprogramming of cells. Am J Cancer Res 2011;1:98-110.
71. Duvivier L, Gerard L, Diaz A, Gillet JP. Linking ABC transporters to the hallmarks of cancer. Trends Cancer 2024;10:124-34.
72. Quazi F, Lenevich S, Molday RS. ABCA4 is an N-retinylidene-phosphatidylethanolamine and phosphatidylethanolamine importer. Nat Commun 2012;3:925.
73. Kitai K, Kawaguchi K, Tomohiro T, Morita M, So T, Imanaka T. The lysosomal protein ABCD4 can transport vitamin B12 across liposomal membranes in vitro. J Biol Chem 2021;296:100654.
74. Gerovac M, Tampé R. Control of mRNA translation by versatile ATP-driven machines. Trends Biochem Sci 2019;44:167-80.
75. Moitra K, Lou H, Dean M. Multidrug efflux pumps and cancer stem cells: insights into multidrug resistance and therapeutic development. Clin Pharmacol Ther 2011;89:491-502.
76. Dong J, Yuan L, Hu C, Cheng X, Qin JJ. Strategies to overcome cancer multidrug resistance (MDR) through targeting P-glycoprotein (ABCB1): an updated review. Pharmacol Ther 2023;249:108488.
77. Amiri-Kordestani L, Basseville A, Kurdziel K, Fojo AT, Bates SE. Targeting MDR in breast and lung cancer: discriminating its potential importance from the failure of drug resistance reversal studies. Drug Resist Updat 2012;15:50-61.
78. Levchenko A, Mehta BM, Niu X, et al. Intercellular transfer of P-glycoprotein mediates acquired multidrug resistance in tumor cells. Proc Natl Acad Sci U S A 2005;102:1933-8.
79. Rafii A, Mirshahi P, Poupot M, et al. Oncologic trogocytosis of an original stromal cells induces chemoresistance of ovarian tumours. PLoS One 2008;3:e3894.
80. Bebawy M, Combes V, Lee E, et al. Membrane microparticles mediate transfer of P-glycoprotein to drug sensitive cancer cells. Leukemia 2009;23:1643-9.
81. Corcoran C, Rani S, O’Brien K, et al. Docetaxel-resistance in prostate cancer: evaluating associated phenotypic changes and potential for resistance transfer via exosomes. PLoS One 2012;7:e50999.
82. Jaiswal R, Luk F, Dalla PV, Grau GE, Bebawy M. Breast cancer-derived microparticles display tissue selectivity in the transfer of resistance proteins to cells. PLoS One 2013;8:e61515.
83. Lv M, Zhu X, Chen W, et al. Exosomes mediate drug resistance transfer in MCF-7 breast cancer cells and a probable mechanism is delivery of P-glycoprotein. Tumour Biol 2014;35:10773-9.
84. Pasquier J, Galas L, Boulangé-Lecomte C, et al. Different modalities of intercellular membrane exchanges mediate cell-to-cell p-glycoprotein transfers in MCF-7 breast cancer cells. J Biol Chem 2012;287:7374-87.
85. Pokharel D, Padula MP, Lu JF, et al. Proteome analysis of multidrug-resistant, breast cancer-derived microparticles. J Extracell Vesicles 2014;3:24384.
86. Zhou H, Zheng Y, Cheng X, et al. Intercellular transfer of P-glycoprotein from the drug resistant human bladder cancer cell line BIU-87 does not require cell-to-cell contact. J Urol 2013;190:1069-75.
87. Cheng XZ, Zhou HL, Tang SX, et al. Intercellular transfer of P-glycoprotein mediates the formation of stable multi-drug resistance in human bladder cancer BIU-87 cells. Biol Open 2019;8:bio041889.
88. Zhang F, Zhu Y, Zhao Q, et al. Microvesicles mediate transfer of P-glycoprotein to paclitaxel-sensitive A2780 human ovarian cancer cells, conferring paclitaxel-resistance. Eur J Pharmacol 2014;738:83-90.
89. Torreggiani E, Roncuzzi L, Perut F, Zini N, Baldini N. Multimodal transfer of MDR by exosomes in human osteosarcoma. Int J Oncol 2016;49:189-96.
90. Wang X, Qiao D, Chen L, et al. Chemotherapeutic drugs stimulate the release and recycling of extracellular vesicles to assist cancer cells in developing an urgent chemoresistance. Mol Cancer 2019;18:182.
91. Jaiswal R, Raymond Grau GE, Bebawy M. Cellular communication via microparticles: role in transfer of multidrug resistance in cancer. Future Oncol 2014;10:655-69.
92. Norris MD, Bordow SB, Marshall GM, Haber PS, Cohn SL, Haber M. Expression of the gene for multidrug-resistance-associated protein and outcome in patients with neuroblastoma. N Engl J Med 1996;334:231-8.
93. Nooter K, de la Riviere GB, Klijn J, Stoter G, Foekens J. Multidrug resistance protein in recurrent breast cancer. Lancet 1997;349:1885-6.
94. Lu JF, Luk F, Gong J, Jaiswal R, Grau GE, Bebawy M. Microparticles mediate MRP1 intercellular transfer and the re-templating of intrinsic resistance pathways. Pharmacol Res 2013;76:77-83.
95. Bouvy C, Wannez A, Laloy J, Chatelain C, Dogné JM. Transfer of multidrug resistance among acute myeloid leukemia cells via extracellular vesicles and their microRNA cargo. Leuk Res 2017;62:70-6.
96. Taylor NMI, Manolaridis I, Jackson SM, Kowal J, Stahlberg H, Locher KP. Structure of the human multidrug transporter ABCG2. Nature 2017;546:504-9.
97. Hou YX, Li CZ, Palaniyandi K, et al. Effects of putative catalytic base mutation E211Q on ABCG2-mediated methotrexate transport. Biochemistry 2009;48:9122-31.
99. Patch AM, Christie EL, Etemadmoghadam D, et al; Australian Ovarian Cancer Study Group. Whole-genome characterization of chemoresistant ovarian cancer. Nature 2015;521:489-94.
100. Ifergan I, Scheffer GL, Assaraf YG. Novel extracellular vesicles mediate an ABCG2-dependent anticancer drug sequestration and resistance. Cancer Res 2005;65:10952-8.
101. Ifergan I, Goler-Baron V, Assaraf YG. Riboflavin concentration within ABCG2-rich extracellular vesicles is a novel marker for multidrug resistance in malignant cells. Biochem Biophys Res Commun 2009;380:5-10.
102. Goler-Baron V, Assaraf YG. Structure and function of ABCG2-rich extracellular vesicles mediating multidrug resistance. PLoS One 2011;6:e16007.
103. Goler-Baron V, Sladkevich I, Assaraf YG. Inhibition of the PI3K-Akt signaling pathway disrupts ABCG2-rich extracellular vesicles and overcomes multidrug resistance in breast cancer cells. Biochem Pharmacol 2012;83:1340-8.
104. Gajos-Michniewicz A, Duechler M, Czyz M. MiRNA in melanoma-derived exosomes. Cancer Lett 2014;347:29-37.
105. Bang C, Thum T. Exosomes: new players in cell-cell communication. Int J Biochem Cell Biol 2012;44:2060-4.
106. Jaiswal R, Gong J, Sambasivam S, et al. Microparticle-associated nucleic acids mediate trait dominance in cancer. FASEB J 2012;26:420-9.
107. Zhuang L, Zhang B, Liu X, et al. Exosomal miR-21-5p derived from cisplatin-resistant SKOV3 ovarian cancer cells promotes glycolysis and inhibits chemosensitivity of its progenitor SKOV3 cells by targeting PDHA1. Cell Biol Int 2021;45:2140-9.
108. Zhu T, Hu Z, Wang Z, et al. microRNA-301b-3p from mesenchymal stem cells-derived extracellular vesicles inhibits TXNIP to promote multidrug resistance of gastric cancer cells. Cell Biol Toxicol 2023;39:1923-37.
109. Wei Y, Lai X, Yu S, et al. Exosomal miR-221/222 enhances tamoxifen resistance in recipient ER-positive breast cancer cells. Breast Cancer Res Treat 2014;147:423-31.
110. Natasha G, Gundogan B, Tan A, et al. Exosomes as immunotheranostic nanoparticles. Clin Ther 2014;36:820-9.
111. Munoz JL, Bliss SA, Greco SJ, Ramkissoon SH, Ligon KL, Rameshwar P. Delivery of functional anti-miR-9 by mesenchymal stem cell-derived exosomes to glioblastoma multiforme cells conferred chemosensitivity. Mol Ther Nucleic Acids 2013;2:e126.
112. Cui X, Chen Y, Zhao L, Ding X. Extracellular vesicles derived from paclitaxel-sensitive nasopharyngeal carcinoma cells deliver miR-183-5p and impart paclitaxel sensitivity through a mechanism involving P-gp. Cell Biol Toxicol 2023;39:2953-70.
113. Ma X, Chen Z, Hua D, et al. Essential role for TrpC5-containing extracellular vesicles in breast cancer with chemotherapeutic resistance. Proc Natl Acad Sci U S A 2014;111:6389-94.
114. Wu S, Luo M, To KKW, et al. Intercellular transfer of exosomal wild type EGFR triggers osimertinib resistance in non-small cell lung cancer. Mol Cancer 2021;20:17.
115. Vella LJ, Behren A, Coleman B, Greening DW, Hill AF, Cebon J. Intercellular resistance to BRAF inhibition can be mediated by extracellular vesicle-associated PDGFRβ. Neoplasia 2017;19:932-40.
116. Ji R, Zhang B, Zhang X, et al. Exosomes derived from human mesenchymal stem cells confer drug resistance in gastric cancer. Cell Cycle 2015;14:2473-83.
117. Qu Z, Wu J, Wu J, Luo D, Jiang C, Ding Y. Exosomes derived from HCC cells induce sorafenib resistance in hepatocellular carcinoma both in vivo and in vitro. J Exp Clin Cancer Res 2016;35:159.
118. Wojtuszkiewicz A, Schuurhuis GJ, Kessler FL, et al. Exosomes secreted by apoptosis-resistant acute myeloid leukemia (AML) blasts harbor regulatory network proteins potentially involved in antagonism of apoptosis. Mol Cell Proteomics 2016;15:1281-98.
119. Kreger BT, Johansen ER, Cerione RA, Antonyak MA. The enrichment of survivin in exosomes from breast cancer cells treated with paclitaxel promotes cell survival and chemoresistance. Cancers 2016;8:111.
120. Lopes-Rodrigues V, Di Luca A, Mleczko J, et al. Identification of the metabolic alterations associated with the multidrug resistant phenotype in cancer and their intercellular transfer mediated by extracellular vesicles. Sci Rep 2017;7:44541.
121. Sullivan R, Maresh G, Zhang X, et al. The emerging roles of extracellular vesicles as communication vehicles within the tumor microenvironment and beyond. Front Endocrinol 2017;8:194.
122. Xing F, Saidou J, Watabe K. Cancer associated fibroblasts (CAFs) in tumor microenvironment. Front Biosci 2010;15:166-79.
123. Richards KE, Zeleniak AE, Fishel ML, Wu J, Littlepage LE, Hill R. Cancer-associated fibroblast exosomes regulate survival and proliferation of pancreatic cancer cells. Oncogene 2017;36:1770-8.
124. Hekmatirad S, Moloudizargari M, Moghadamnia AA, et al. Inhibition of exosome release sensitizes U937 cells to PEGylated liposomal doxorubicin. Front Immunol 2021;12:692654.
125. Muralidharan-Chari V, Kohan HG, Asimakopoulos AG, et al. Microvesicle removal of anticancer drugs contributes to drug resistance in human pancreatic cancer cells. Oncotarget 2016;7:50365-79.
126. Federici C, Petrucci F, Caimi S, et al. Exosome release and low pH belong to a framework of resistance of human melanoma cells to cisplatin. PLoS One 2014;9:e88193.
127. Atai NA, Balaj L, van Veen H, et al. Heparin blocks transfer of extracellular vesicles between donor and recipient cells. J Neurooncol 2013;115:343-51.
Cite This Article

How to Cite
Yang, A.; Sun H.; Wang X. Intercellular transfer of multidrug resistance mediated by extracellular vesicles. Cancer. Drug. Resist. 2024, 7, 36. http://dx.doi.org/10.20517/cdr.2024.84
Download Citation
Export Citation File:
Type of Import
Tips on Downloading Citation
Citation Manager File Format
Type of Import
Direct Import: When the Direct Import option is selected (the default state), a dialogue box will give you the option to Save or Open the downloaded citation data. Choosing Open will either launch your citation manager or give you a choice of applications with which to use the metadata. The Save option saves the file locally for later use.
Indirect Import: When the Indirect Import option is selected, the metadata is displayed and may be copied and pasted as needed.
About This Article
Special Issue
Copyright
Data & Comments
Data
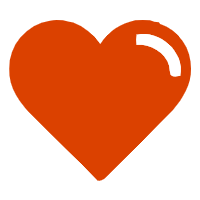
Comments
Comments must be written in English. Spam, offensive content, impersonation, and private information will not be permitted. If any comment is reported and identified as inappropriate content by OAE staff, the comment will be removed without notice. If you have any queries or need any help, please contact us at support@oaepublish.com.