HDAC-driven mechanisms in anticancer resistance: epigenetics and beyond
Abstract
The emergence of drug resistance leading to cancer recurrence is one of the challenges in the treatment of cancer patients. Several mechanisms can lead to drug resistance, including epigenetic changes. Histone deacetylases (HDACs) play a key role in chromatin regulation through epigenetic mechanisms and are also involved in drug resistance. The control of histone acetylation and the accessibility of regulatory DNA sequences such as promoters, enhancers, and super-enhancers are known mechanisms by which HDACs influence gene expression. Other targets of HDACs that are not histones can also contribute to resistance. This review describes the contribution of HDACs to the mechanisms that, in some cases, may determine resistance to chemotherapy or other cancer treatments.
Keywords
INTRODUCTION
Much progress has been made in the detection and treatment of cancer. Unfortunately, there are still several key problems to be solved, including very aggressive specific cancers, metastasis, and resistance to therapy[1,2]. Cancer therapy involves multiple strategies and approaches, from surgery to immunotherapy. Chemotherapy is still part of several protocols in cancer treatment or adjuvant therapies[3]. Several chemotherapeutic agents work by damaging essential cell functions or triggering uncontrollable cell stress, leading to cell death[4]. All these agents have certain limitations due to their toxicity to non-tumor cells and the emergence of resistance[5]. Drug resistance is one of the main causes of failure of cancer therapies and can also lead to multidrug resistance (MDR)[2]. The first findings on resistance go back to Luria and Delbruck, who described that genetic mutations in bacteria make them resistant to viral infection[6]. Goldie and Coldman later transferred this concept to the resistance of cancer to therapeutic drugs, which is due to random mutations in cells[7].
Depending on the mechanism, chemoresistance can be intrinsic, if it occurs before treatment due to genetic and epigenetic factors, or acquired, if it arises during treatment in previously susceptible tumor cells[8]. Various studies have described different molecular mechanisms that play a role in drug resistance: transport pumps, DNA damage and repair, oncogenes and tumor suppressor genes, epithelial-mesenchymal transition (EMT), etc. In this review, histone deacetylases (HDACs) are discussed with a focus on class I and IIa and their involvement in the development of mechanisms of drug resistance. Regulation of lysine acetylation turnover by HDACs can affect various cellular responses, including drug resistance phenotypes. Resistance can be influenced by changes in gene expression, which is determined by the control of chromatin accessibility (epigenetics) or, for example, by the activity of transcription factors (TFs) that are subject to acetylation. In addition, other biological functions not related to gene expression may also be under the control of lysine acetylation turnover. Importantly, a repertoire of different HDAC inhibitors (HDACi) is available that may counteract HDAC-induced drug resistance.
THE HDAC WORLD IN BRIEF
Acetylation is an ancient and very simple post-translational modification (PTM) of amino acids, but its effects on cell fate are impressive. Two enzyme families act antagonistically in the control of lysine acetylation: the lysine acetyltransferases (KATs) and the lysine deacetylases (KDACs). As histones are important and well-studied substrates for these enzymes, they are also referred to as histone acetyltransferases (HATs) and HDACs. In this chapter, we would like to give a brief introduction to the world of HDACs and highlight some important features.
HDACs represent a heterogeneous family of 18 enzymes that can be divided into five different classes/subclasses[9,10]. They are responsible for removing the acetyl group but also other small hydrophobic acyl groups, such as formyl, propionyl, butyryl, malonyl, succinyl, glutaryl, crotonyl, and 2-hydroxyisobutyryl from ε-amino group of lysines[9,11,12]. The first distinction, based on the catalytic mechanism, the required cofactor, and the structure of the catalytic pocket, is between zinc- or metal-dependent and NAD+-dependent enzymes[13].
Eleven are the metal-dependent HDACs, which are divided into three classes based on sequence and structural homologies. Class I includes HDAC1, 2, 3, and 8, which are largely nuclear enzymes. HDAC1 and HDAC2 are much closer to each other and often coassemble in different repressive complexes[14]. Like HDAC1 and 2, HDAC3 forms multiprotein complexes that are important for the maturation of full catalytic activity. HDAC8 is the more distant member and does not need to assemble into protein complexes to develop full catalytic activity. HDAC1, 2, or 3 often has a strong influence on cell proliferation[15,16].
HDAC4, HDAC5, HDAC7, and HDAC9 form class IIa, which is distinct from class IIb. Common features of class IIa are the intense regulation of nuclear-cytoplasmic shuttling by phosphorylation and the acquisition of the Tyr/His mutation in the catalytic pocket in vertebrates. This amino acid substitution minimizes/abrogates KDAC activity. In addition, class IIa HDACs show the presence of an extensive amino-terminal region dedicated to protein interaction, including the binding of co-repressors and TFs [Figure 1], of which the members of the myocyte enhancer factor 2 (MEF2) family are best characterized[17]. In vertebrates, class IIa shows very low deacetylase activity toward acetyl-lysines, but their ability to bind with repressive class I complexes allows deacetylase activity in trans[18,19]. For this reason, it has been proposed that class IIa acts as an acetyl-lysine reader[20-22]. Class IIa, apart from HDAC7, also possesses a glutamine-rich domain that enables homo-/heterodimerization [Figure 1] and still unexplored influences on chromatin structure[23].
Figure 1. AlphaFold prediction of Class I and Class IIa structures discussed in this review (Q13547; Q92769; O15379; P56524; Q9UQL6. Q8WUI4; Q9UKV0). The different views were similarly oriented, taking as reference the eight-stranded parallel β-sheet of the common deacetylase domain. The colors indicate the different per-residue confidence scores (pLDDT). Some regions below 50 pLDDT may be unstructured in isolation. For details, see https://alphafold.ebi.ac.uk. pLDDT: Predicted Local Distance Difference Test.
Class IIb essentially comprises the cytosolic enzymes HDAC6 and HDAC10. HDAC6 has several target proteins that are not histones, such as α-tubulin and Hsp90, and can regulate their stability by deacetylation[24,25]. In contrast, HDAC10 is a polyamine deacetylase with a very high substrate specificity. The structure of the active site is specific for the hydrolysis of N8-acetylspermidine and disfavors the hydrolysis of acetyl lysine[26,27].
The NAD+-dependent HDACs are known as sirtuins. They form class III and are not discussed in this overview. Finally, HDAC11 alone constitutes the class IV. HDAC11 is a potent lysine defatty-acylase rather than a deacetylase and makes important contributions to the regulation of the immune system.
For a discussion of the general and specific aspects of the various HDACs, we refer the reader to previously published reviews[9,10,15,22,28-31].
In summary, zinc-dependent HDACs 1, 2, 3, which are part of various multiprotein complexes and, in some cases, interact with class IIa HDACs, serve as master regulators of histone acetylation and strong candidates for modulating epigenetic resistance to anticancer therapies. Given that these HDACs are components of different multiprotein complexes whose composition - both in terms of stoichiometry and individual components - varies depending on the neoplastic context, HDACs can either promote drug resistance or act antagonistically. Each of these aspects requires detailed investigation.
RESISTANCE TO CHEMOTHERAPY: CISPLATIN
Cisplatin is a metal-based chemotherapeutic agent used to treat various types of cancer[32,33]. Cisplatin binds to DNA and leads to the formation of DNA-platinum adducts, which initiate the DNA damage response (DDR)[33,34]. The efficacy of this approach decreases over time due to the emergence of drug resistance[32,33]. Resistance to cisplatin can arise through many different mechanisms[35]. Recently, class I HDACs have also been found to be responsible for cisplatin resistance/sensitization. In MCF7 breast cancer cells, TRIM46-dependent degradation of HDAC1 enhances the expression of genes involved in both DNA replication and DNA repair[36]. Proteasomal-dependent degradation of HDAC1 increases the expression of genes involved in DNA repair, such as breast cancer 1 gene (BRCA1)[36], which is involved in chemoresistance to various drugs[36-38]. The chemosensitivity of cisplatin is also regulated in laryngeal cancer cells by HDAC1 via the modulation of interleukin-8 expression[39]. Differently, in non-small cell lung cancer (NSCLC), an epigenetic mechanism under HDAC1 control regulates cisplatin resistance by suppressing the expression of ornithine decarboxylase antizyme 1 (OAZ1)[40]. OAZ1 triggers the degradation of ornithine decarboxylase and suppresses the synthesis of polyamines[41], but how this leads to resistance remains to be clarified. The role of HDAC2 in chromatin remodeling at the site of DNA lesions, which could contribute to the regulation of chemoresistance, is interesting but remains poorly understood[18,42,43].
Surprisingly, the role of HDAC3 in cisplatin resistance is not well understood[44], but its contribution when complexed with class IIa should be considered, and indeed, some reports have suggested a role for HDAC4 in regulating cisplatin resistance. Stronach et al. have described that ovarian cancer cells resistant to cisplatin have upregulated expression of both HDAC4 and STAT1 and that downregulation of HDAC4 leads to platinum sensitization, increased caspase activation and apoptosis. In addition, HDAC4 is overexpressed in clinical samples that exhibit platinum resistance. HDAC4 and STAT1 can interact through co-immunoprecipitation, and HDAC4 deacetylates STAT1, causing its phosphorylation at Y701. This regulation triggers the nuclear translocation of STAT1 and the activation of its transcriptional program, leading to platinum resistance[45]. Further confirmation using additional methods such as mass spectrometry should help to validate this interaction further. Since HDAC3 is the predominant HDAC4 partner conferring enzymatic activity to the complex, its silencing in the regulation of STAT1- and HDAC4-dependent deacetylation should be investigated.
In gastric cancer cells, analysis of the cisplatin pangenome response identified HDAC4 as the major repressed epigenetic regulator. HDAC4 inhibits the cytotoxicity of cisplatin and favors chemoresistance. Its expression is high in gastric tumors compared to healthy tissue, while HDAC4 mutations correlate with a good prognosis[46]. This effect is thought to be partially dependent on TP53[46]. Furthermore, the involvement of HDAC4 in the regulation of H2K120ac status at the site of DNA lesions, as well as its influence on the efficiency of homology-directed repair via HDAC2, may also contribute to this resistance[18].
Mutations in TP53 that promote cell apoptosis, senescence, and cell cycle arrest and are associated with platinum chemoresistance are common in ovarian cancer[47,48]. Reintroduction of TP53 into the ovarian adenocarcinoma cell line SKOV-3, which is characterized by its deletion, leads to upregulation of pro-apoptotic proteins and reduces cis-platinum resistance[49]. Importantly, in this context, TP53 induction decreased HDAC4 expression while also increasing HDAC4 phosphorylation and its accumulation in the cytoplasm - both of which diminish its repressive influence. As a result, an increase in total histone 3 acetylation was observed[49]. Although the relationships between HDAC4 and HIF-1a have not been fully explored, Zhang et al. reported that they form a complex that regulates autophagy and apoptosis controlled by TP53[49,50]. In ovarian and lung cancer cells, this complex is crucial for cis-platinum resistance. Mechanistically, overexpression of HIF-1a inhibits ATG12 and BAX, but enhances BCL2 expression. Autophagy is directly mediated by HDAC4 through deacetylation of the TF CREBZF, which is responsible for the transcription of ATG2 and possibly ATG12. Under normoxic conditions, TP53 leads to a massive decrease in HIF-1a, while expression of HDAC4 and HIF-1a leads to cis-platinum resistance and worsened overall survival in ovarian cancer patients[49].
Another example of the involvement of class IIa HDACs in cisplatin resistance in ovarian cancer is the tumor suppressive role of intermediate filament family orphan 1 (IFFO1). IFFO1 plays an important role in the regulation of tumor progression and immune infiltration[51,52]. It is poorly expressed in ovarian cancer tissue, as well as other cancer tissues, and cisplatin-resistant cells are characterized by a low IFFO1 level. The regulatory mechanism of IFFO1 depends on the interaction between HDAC5 and the TF YY1. HDAC5 binds to the IFFO1 promoter via YY1, leading to its deacetylation and a reduction in transcription[53]. Since the interaction between YY1 and HDAC5 was observed also in other studies[54-56] and HDAC5 expression is altered in ovarian cancer, this result is of particular relevance[57]. Figure 2 summarizes the effects and mechanisms of class I and IIa HDACs that may influence the response of cancer cells to treatment with cisplatin.
Figure 2. Summary of reported roles and mechanisms of class I and class IIa HDACs in different cancer cell lines that respond to treatment with cisplatin. Mechanisms of resistance are shown in purple, while mechanisms of susceptibility are shown in light orange. The relationships between HDAC4 and TP53 are poorly defined (question marks). In addition, the existence of different multiprotein complexes with class I and IIa HDACs is also considered. BIK is a BH3-only pro-apoptotic BCL2 family member. For details, please refer to the text. HDACs: Histone deacetylases; BIK: BCL2 interacting killer; BCL2: B-cell CLL/lymphoma 2.
OTHER GENOTOXIC DRUGS
Resistance to other genotoxic drugs has also been reported to depend on class I and IIa HDACs. In multiple myeloma (MM), inhibition of HDAC1 leads to a re-sensitization of cells to the proteasome inhibitor bortezomib (BTZ) and to a reduction in DNA repair potential, thus potentially increasing the susceptibility to genotoxic stress. The heterochromatin protein 1 (HP1) family α, β, and γ are readers of H3K9me2/3[58]. HP1α and β are distributed along heterochromatic regions (centrosomes and telomeres), while HP1γ is associated with both euchromatin and heterochromatin, in regions with actively transcribed genes that play a role in transcriptional elongation[59]. HP1γ acts as a platform for histone modifiers in association with HDAC1[60]. In MM, HDAC1 interacts directly with the protein HP1γ and deacetylates it at Lys 5 to improve the stability of the protein and its nuclear condensation. This improves DNA repair by forming a complex with MDC1 and promotes chromosomal accessibility of genes that determine MM cell survival and BTZ resistance[61]. A mechanism that should also be evaluated for the response to other genotoxic treatments.
Doxorubicin is an anthracycline antibiotic that acts by inhibiting topoisomerase IIa (TOPO2A), thereby causing an accumulation of double-strand breaks (DSBs). It was first isolated from Streptomyces peucetius by Arcamone in 1969[62]. Resistance to doxorubicin is mainly related to the overexpression of ABC efflux pumps. Other mechanisms include increased expression of TOPO2A or anti-apoptotic BCL2 proteins[63]. Reduced oxygen species (ROS) and a change in redox activity/status may also promote resistance to doxorubicin as well as other chemotherapeutic agents[64].
A non-epigenetic role of HDAC3 in acute myeloid leukemia (AML) responsible for resistance to anthracyclines and cytarabine has been reported. Deletion or inhibition of HDAC3 impairs DNA repair, which occurs mainly via the AKT pathway[65]. This pathway is involved in cell survival and proliferation in leukemia, where it is often activated and associated with a poor prognosis[66]. Increased AKT signaling is associated with the onset of chemoresistance[67]. Treatment with Ara-C in combination with doxorubicin leads to increased expression of HDAC3, which interacts with AKT and deacetylates it at the level of Lys 20. This modification leads to its phosphorylation and increased activity[65]. Through this mechanism, AML cells activate the resolution of DNA damage. Finally, treatment with the HDAC3 inhibitor (RGFP966) reverses Ara-C and doxorubicin resistance[65].
In lung cancer-derived cells with exogenous overexpression of cancer-regulated gene 2 (CUG2), there is upregulation of EGFR and consequent resistance to doxorubicin treatment due to increased expression of antioxidant proteins such as MnSOD, Foxo1, and Foxo4 and the MDR genes MRP2 and BCRP. EGFR causes the activation of STAT1, a known factor of doxorubicin resistance, which was downregulated by the non-selective HDACs inhibitor TSA thanks to increased acetylation[68]. HDAC4 is highly expressed in CUG2 cells, and downregulation of HDAC4 leads to doxorubicin sensitization through a decrease in antioxidant proteins and increased apoptosis[69].
ANTIMETABOLITES
Antimetabolites interfere with tumor growth by inhibiting pyrimidine and purine biosynthesis, which leads to a blockade of DNA synthesis and triggers DNA strand breaks and apoptosis in highly proliferating cancer cells[70]. Antimetabolites are divided into purine analogs (6-mercaptopurine), pyrimidine analogs [5-fluorouracil (5-FU), gemcitabine, azacitidine], and antifolates (methotrexate). Azacitidine and its derivatives are epigenetic drugs that inhibit DNA methyltransferases (DNMT)[71]. In addition to the classical resistance mechanisms, antimetabolites can cause an overexpression of thymidylate synthase, an accumulation of deoxyuridine monophosphate, and a downregulation of 5,10-methylenetetrahydrofolate[72]. Gemcitabine resistance is associated with changes in proteins involved in its metabolism[73]. Methotrexate resistance is caused by changes in dihydrofolate reductase and retention of the drug in the intracellular space[74].
5-FU is the main therapy for colorectal cancer (CRC). Unfortunately, patients often became resistant to this treatment[75,76]. Dihydropyrimidine dehydrogenase (DPD) can rapidly degrade 5-FU and confer resistance[77]. The sphingosine kinase SphK2 produces sphingosine-1-phosphate (S1P) in the cell nucleus, which has been shown to inhibit the activities of Hdac1 and 2[78]. High SPHK2 expression in CRC cell lines promotes an overall increase in H3K56 acetylation as determined by whole genome analysis. The increase in H3K56ac also affects an exon of DPD and correlates with its increased expression. Therefore, HDAC1 and 2 repress the transcription of DPD and the degradation of intracellular 5-FU. In this context, HDAC1 and 2 are considered epigenetic regulators that counteract resistance to 5-FU[79]. However, contrary results have also been reported in various contexts. Resistance to 5-FU was observed in the CRC cell line RKO, which is negative for HDAC2. This benefit was associated with a higher efficiency of ATM signaling and possibly enhanced DNA repair activity, but with an undefined mechanism[80]. Studies on class IIa and 5-FU resistance date back to 2010, when it was reported that HDAC7 affects HIF-1a-induced resistance to 5-FU-induced apoptosis in lung cancer cells[81]. In osteosarcoma, miR-140 has been reported to contribute to chemoresistance to methotrexate and 5-FU. The proposed mechanism involves suppression of HDAC4 expression and consequent cell cycle arrest in G1 and G2 with a reduced proliferation rate[82]. Other studies have also indicated a contribution of HDAC4 to 5-FU resistance in CRC[83]. In summary, although there is some evidence for a possible contribution of class IIa to 5-FU resistance, the mechanisms involved still need to be clarified and further studies are required.
HDAC4, together with HDAC7, may also contribute to resistance to gemcitabine in pancreatic ductal adenocarcinoma (PDAC). Knockdown (KD) of these two HDACs enhances gemcitabine cytotoxicity and apoptosis both in vitro and in vivo in mouse models of PDAC. Interestingly, this activity is caused by MARK2-dependent phosphorylation and the cytosolic accumulation of deacetylases. As discussed below, this cytosolic accumulation of class IIa HDACs also influences resistance to taxanes[84].
TAXANES
Taxanes are a class of chemotherapeutic agents that influence microtubule dynamics. In highly proliferating cells, they primarily cause mitotic arrest and, ultimately, cell death by mitotic catastrophe[85]. The taxanes include paclitaxel (PTX), docetaxel (DTX), and cabazitaxel. They are often used to treat metastatic breast cancer, non-small lung cancer, prostate, ovarian and bladder cancer. PTX and DTX bind to a hydrophobic cleft in β-tubulin[86]. PTX is the most used drug for the treatment of triple-negative breast cancer (TNBC)[87,88]. Patients may develop resistance through changes in cell survival, changes in tubulin, such as mutations that reduce binding to PTX, PTMs, or increased expression of MDR efflux transporters[89,90].
In NSCLC, HDAC1 is critical for resistance to PTX[91]. HDAC1 can form a complex with SIN3A, a core component of the histone deacetylation activity-associated transcriptional repressor complex[92], and C3b, the active fragment of complement component 3 (C3)[93,94]. This repressive complex controls the downregulation of the antiproliferative gene GADD45D[95] by histone deacetylation and thus leads to PTX resistance[96].
The involvement of HDAC1 in the maintenance of resistance to taxanes has been confirmed for DTX and again for NSCLC, but the proposed mechanism is different and involves miR-200b. Of interest is the definition of the epigenetic switches that control HDAC1 transcription. Here, HDAC1 is repressed after H3K27me3 modification of its promoter by SUZ12, the catalytic component of polycomb repressive complex 2 (PRC2)[97-99]. HDAC1 suppression leads to an increase in miR-200b levels, which are known to reverse DTX resistance[100]. SUZ12 has been reported to be recruited to the HDAC1 promoter by the lncRNA MARCKSL1-2[99]. Interestingly, a contribution of HDAC3 to taxane resistance has also been observed in some studies[101,102]. It will be important to study whether a complex among HDAC3 and class IIa HDACs is required to confer resistance.
Using a single-guide RNA screen to assess genes involved in PTX resistance, class IIa HDAC9 and its truncated amino-terminal isoform MEF-2 interacting transcription repressor (MITR) were identified among the top hits[103]. MITR is highly expressed in the PTX-resistant TNBC cells MDA-MB-231, and KD of MITR results in an impressive reduction in the IC50 of PTX compared to control cells or cells with HDAC9 KD. This outcome likely reflects the relative abundance of the two isoforms in this cell line. Overexpression of MITR enriches the JAK/STAT3 pathway and leads to an increase in IL11 production. Interestingly, silencing IL11 abrogates PTX resistance in MITR-overexpressing cells. Mechanistically, MITR interacts with MEF2A and abolishes its repressive influence on the IL11 promoter[104]. The mechanisms underlying MITR’s influence on MEF2A and IL11 expression are unclear, as is the potential involvement of other HDACs. This hypothesis requires further studies.
Microtubule affinity-regulating kinases (MARK1-4) are important regulators of cell polarity and cell division. They are upregulated in various pathological conditions such as cancer[105,106]. High MARK2 expression correlates with poor prognosis in patients with pancreatic cancer and with PTX resistance. In response to PTX treatment, MARK2 phosphorylates class IIa HDACs (mainly HDAC4 and HDAC7 in this study) and promotes their cytoplasmic localization. PTX resistance of MARKs depends on the phosphorylation of HDAC4/7, which in turn stimulates YAP activity by modulating the LATS2/YAP complex. KD of HDAC4 and HDAC7 abolishes PTX resistance in PANC-1 cells[84]. Additional mechanisms have been proposed for the regulation of DTX resistance by HDAC4 based on the suppression of H3 acetylation of the miR-200b promoter[100].
HORMONE THERAPY
Hormone therapy uses the natural function of hormones as chemical messengers produced by endocrine organs to treat hormone-dependent cancers such as breast, prostate, and ovarian cancer. It includes hormone analogs, inhibitors of hormone receptors or hormone synthesis[107]. The first hormonal agent used in clinical trials was tamoxifen[108,109]. Endocrine therapy, especially the estrogen antagonist tamoxifen, has been shown to improve the survival of patients with estrogen receptor-alpha-positive breast cancer. However, the occurrence of tamoxifen resistance is an increasing obstacle to the effectiveness of the therapy. Endocrine resistance is mainly due to aberration of estrogen/progesterone receptor signaling pathways, PTMs, epigenetic alterations of estrogen receptor 1 (ESR1), increased signaling through the tyrosine kinase receptor, and alteration of cell cycle progression[110].
Epigenetic remodeling may be responsible for resistance to endocrine therapies[111], and several studies have shown that HDACi can reverse such resistance[112-115]. However, in some clinical trials with different HDACi and exemestane (AI-aromatase inhibitor), contrasting results were obtained, with an improved survival rate not always observed[116]. In addition, repressive HDAC complexes can associate with estrogen receptors (ERs) and contribute to their transcriptional output[117-125].
To solve this problem, Zhou et al. developed an AI-resistant cell model and found that downregulation of serine protease inhibitor serpin family A member 3 (SERPINA3), a target gene of ESR1, induces endocrine resistance[126]. Its downregulation increases the expression levels of ankyrin repeat domain containing 11
Of particular interest is that impairment of HDAC1/2 can cause basal-like cells to adopt a luminal A-cell phenotype with increased ER expression and increased sensitivity to tamoxifen treatment[131].
The TF, stem cell-related SOX9, is responsible for tamoxifen resistance, tumor invasion, and metastasis[132,133]. One of the regulators of SOX9 is SIRT1. It causes SOX9 deacetylation, which is required for its increased localization in the nucleus. SOX9 is localized in the nucleus in ER+ breast cancer cells, and its level is increased in cells resistant to tamoxifen[130]. Similarly, the deacetylase domain of HDAC5 physically interacts with SOX9 via its HMGB domain and triggers its deacetylation and nuclear localization. This leads to tamoxifen resistance thanks to the SOX9 transcription program[130]. In addition, HDAC5 is regulated by C-MYC, which directly controls its expression in breast cancer cells. C-MYC is a known trigger of tamoxifen resistance in breast cancer[134]. Consequently, switching off HDAC5 significantly reduces tamoxifen resistance[129]. Similarly, HDAC9 has been associated with tamoxifen resistance. Its expression correlates with poor response to 4-hydroxy-tamoxifen (OHTam) and poorer prognosis in patients treated with OHTam. Its ectopic overexpression reduces ESR1 mRNA and protein levels and inhibits its transcriptional activity[135]. In contrast, one report observed a pro-tamoxifen effect of HDAC4 in ER-positive MCF-7 and T47D cell lines. However, the mechanism by which HDAC4 directs such activity remains unclear[136].
Androgen deprivation therapy (ADT) is the most important treatment for locally advanced and metastatic prostate cancer. Unfortunately, resistance very often occurs within a few years, leading to castration-resistant prostate cancer (CRPC)[137]. Multiple different mechanisms of alteration are engaged to strongly sustain the AR signaling and CRPC[138].
The involvement of HDACs in ADT resistance has been described in several studies, and treatments with HDACi have been proposed to overcome drug resistance in CRPC[139]. One study demonstrated that the expression of androgen receptor (AR)-regulated genes is dependent on HDAC1 or HDAC3[140], a finding later confirmed by another study[141]. Furthermore, it has been proposed that the activities of class I HDACs are necessary for the assembly of the coactivator/RNA polymerase II complex after AR binds to the enhancers of target genes[140].
The orphan nuclear receptor TLX (NR2E1) is upregulated in prostate cancer, especially in metastatic CRPC. TLX can also induce resistance to androgen deprivation by suppressing AR transcription. HDAC1 and HDAC3 are important to propel this suppression[142]. To make the androgen response even more complex, HDAC3 can also suppress AR expression through the involvement of FOXO1[143].
A functional screen identified eight candidate genes for AR-directed therapy resistance, which are mutated by APOBEC3B, including HDAC5[144]. Cytosine deaminase APOBEC is an important trigger of mutations in cancer. In prostate cancer, the synaptotagmin-binding cytoplasmic RNA-interacting protein (SYNCRIP), which suppresses APOBEC-dependent mutagenesis, is frequently lost. Its absence leads to resistance to AR-targeted therapies. Knockout (KO) of HDAC5 has revealed that this class IIa HDAC is involved in maintaining resistance to the AR antagonist enzalutamide in prostate cells lacking SYNCRIP[144]. However, the precise mechanism by which HDAC5 contributes to this resistance, and the impact of the associated mutations, remains unclear. Considering the previous observation that HDAC4 can antagonize AR activity through SUMOylation, a similar contribution could be evoked for HDAC5. However, HDAC5 was much less efficient in supporting AR SUMOylation[145]. Nevertheless, these results are of interest and warrant further investigation.
TARGET-DIRECTED THERAPY
Cancer cells are dependent on the dysregulation of certain signaling pathways for their vigorous growth and aggressiveness. In some cases, specific inhibitors have been developed, but resistance and relapse often occur after an initial positive response[146-148]. In the following, we discuss some examples of the involvement of HDACs in the resistance mechanism.
Among pathway inhibitors, MAPK kinase inhibitors and MEKi-based therapies have received FDA approval for some cancers[149,150]. The RAS/RAF/MEK/ERK signaling pathway [mitogen-activated protein kinase (MAPK)] is associated with tumor cell proliferation and survival, particularly in cancers that harbor BRAF and/or RAS mutations[151].
Trametinib is a selective inhibitor of MEK1/2, which prevents RAF- and RAS-dependent MEK phosphorylation and thus inhibits ERK phosphorylation[151]. HDAC3 is thought to be involved in MEKI resistance in PDAC[149]. The NCor1/HDAC3 complex interacts with G9a, a Lys methyltransferase, and SETD5, a chromatin-associated protein with a catalytic methyltransferase SET domain, which instructs the complex to deacetylate H3K9ac, resulting in this Lys residue methylation at genes involved in MEKI resistance, such as PDK4[152]. The authors combined the treatment of HDAC3i and G9ai in trametinib-resistant cells, which restored MEKi sensitivity[149].
Trametinib resistance has also been identified in NSCLC, and HDAC3 inhibition provides therapeutic benefits in a Kras-mutated/Lkb1-depleted mouse model of NSCLC[153].
Other pathway inhibitors that have been studied in the context of HDACs-mediated resistance are tyrosine kinase inhibitors (TKIs), such as sunitinib, which acts as an anti-angiogenic agent and is used in particular for kidney cancer[154]. Resistance to these inhibitors could be due to proangiogenic signaling pathways, changes in the tumor microenvironment, increased tumor invasion and metastasis, microRNA-mediated drug resistance, or activation of other signaling pathways[155].
ANXA1 is linked to sensitivity to TKIs[156], and in clear cell renal cancer (CCRC), YTHDC1, a reader of the m6A modification[157], interacts with ANXA1 mRNA via the m6A modification, reducing its stability, thereby inactivating the MAPK signaling pathway and rendering CCRC cells sensitive to TKIs[158]. HDAC2 in complex with TF YY1 suppresses the expression of YTHDC1, indirectly stabilizes ANXA1 mRNA, activates the MAPK pathway, and induces TKI resistance[158].
Sorafenib is a multitarget TKI approved for the treatment of hepatocellular carcinoma (HCC). Sorafenib targets the RAF/MEK/ERK signaling pathway and receptor tyrosine kinases and inhibits tumor growth and progression[159]. A current problem of HCC patients is the development of sorafenib resistance, which occurs through different mechanisms, including epigenetics mechanisms, transport processes regulation, modulation of cell death, and alterations in the tumor microenvironment[160,161]. The TF MEF2D is one of the main partners of HDAC4[162,163]. It has been described that the influence of HDAC4 on sorafenib resistance depends on MEF2D. This TF is highly expressed in HCC patients with poor prognosis and is more abundant in sorafenib-resistant cells than in sensitive cells. HDAC4 forms a complex with MEF2D and directly regulates its activity on SPRY4, an inhibitor of the MAPK/ERK signaling pathway. The MAPK/ERK signaling pathway is a known player in sorafenib resistance[164,165]. Treatment with the HDAC4 inhibitor tasquinimod inhibits MEF2D-dependent suppression of SPRY4 and leads to re-sensitization to sorafenib in a liver cancer mouse model[166]. Of course, one must bear in mind that tasquinimod also has other targets and that specific activity against HDAC4 cannot be ruled out[167].
PALBOCICLIB
Palbociclib is a CDK4/6 inhibitor that is used in particular for the treatment of hormone-positive breast cancer. It inhibits the phosphorylation of the tumor suppressor gene RB and leads to cell cycle arrest. Resistance to palbociclib is an emerging problem caused both by a failure of the CDK4/6-RB axis and by changes in the upstream regulatory factors[168,169]. It is known that RB binds to HDAC1 via its pocket domain and an LXCXE motif in HDAC1[170]. Phosphorylation-dependent recruitment of HDAC1/2 is also utilized by RB to form a repressive complex[171]. Based on this historical evidence, one could argue that HDAC1/2 is necessary for the antiproliferative effect of palbociclib - a hypothesis that has not yet been investigated. Interestingly, enhancing the repressive activity of the RB1/TEAD4/HDAC1 axis leads to the suppression of DNA repair genes and sensitizes the cells to oxaliplatin treatment[172]. HDAC5 has also been reported to interact with the RB protein, and binding is reduced by CDK4/6-dependent phosphorylation and enhanced by palbociclib treatment. HDAC5 acts as a repressive arm of RB-dependent gene silencing by inhibiting H3K27ac at multiple oncogenic loci associated with the cell cycle[173]. In prostate and breast cancer cells, HDAC5 KD promotes resistance to palbociclib. Indeed, palbociclib is not sufficient to activate HDAC5-dependent RB inhibitory activity in HDAC5 KD cells, but concurrent treatment with NEO3724, a dual BET-CBP/p300 inhibitor, restores sensitivity to palbociclib in cancer cells and xenografts[173]. Of note, all class IIa HDACs, but not class I HDACs, appear to be involved in palbociclib-dependent inhibition of H3K27ac at cell cycle loci in prostate cancer cells. Zhou et al. also reported that the expression of HDAC5 is frequently reduced in various solid tumors, including breast and prostate cancer[173]. This observation is inconsistent with several reports indicating a positive correlation between class IIa HDACs and cancer aggressiveness. However, we need to consider the contextual influence that could explain the differential contribution of these epigenetic regulators to tumorigenesis in specific contexts. The author also used the inhibitor LMK235 to specifically demonstrate the contribution of HDAC5. However, this compound shows some preference but no specificity toward HDAC5[174,175].
PARP INHIBITORS
PARP inhibitors (PARPi) are effective in the treatment of ovarian and breast cancer, especially in cancers that are deficient in homologous recombination (HR) and DNA repair mechanisms due to BRCA1/2 mutations[176]. Nevertheless, cancer cells often develop resistance to PARPi, which is why combination therapies need to be developed[177,178].
The influence of class I HDACs on DSB repair by HR suggests a possible contribution of these deacetylases to the efficacy of PARPi treatments[18,179,180]. In some cases, the contribution of specific class I inhibitors has been explored with positive results in preclinical models of ovarian cancer and one study suggested a new role for HDAC2 in the regulation of splicing of the HR genes BRCA1/FANC[181,182].
Class IIa HDACs can also affect DNA repair pathways and HR. Although the detailed mechanisms remain to be fully explored, an effect through the binding of members of the MEF2 family or through the regulation of chromatin accessibility in complex with class I HDACs has been proposed[18,183-185].
Class IIa HDACs interact with MEF2 at the level of the N-terminal domain within the nucleus when HDACs IIa members are not phosphorylated at 14-3-3 binding sites[8,22,186]. Salt-inducible kinase 2 (SIK2), an AMPK-related protein kinase that has a positive effect on ovarian cancer progression, phosphorylates HDACs IIa, causing its cytoplasmic localization and inhibiting its binding to MEF2[187,188]. The SIK2 inhibitors ARN3236 and ARN3261 prevent HDAC4/HDAC5 phosphorylation, resulting in their nuclear localization and inhibition of the MEF2D transcriptional program. ARN3236 and ARN3261 were found to repress transcription of DNA repair genes such as EXO1, FANCD1, and XRCC4 due to inhibition of MEF2D by class IIa HDACs and restore PARPi sensitivity in ovarian cancer and TNBC cells[178].
REVERSAL OF RESISTANCE: NEW PERSPECTIVES FOR HDAC INHIBITORS
A logical perspective in defining the contribution of HDACs to drug resistance is their targeting by specific inhibitors. The first frontier stems from biology. Our current knowledge of the contribution of HDACs to resistance is not clear and adverse effects cannot be excluded. We must assume that under certain circumstances, these enzymes play a role in determining the effect of drug treatment. The second limit concerns inhibitors. We have had experience with HDACi for more than two decades, but the clinical applications are few and limited to a pair of hematologic cancers[9]. The first generation of non-selective HDACi has failed in several clinical trials alone or in combination. The major problems are lack of selectivity, various off-targets, and toxicity. These non-selective HDACi include clinically approved drugs such as vorinostat/SAHA, romidepsin/FK228, belinostat PXD101, and panobinostat/LBH589. They act as zinc chelators by penetrating the catalytic pocket of HDACs[189]. The efficacy of these HDACi in the treatment of solid tumors as single agents was disappointing[190,191]. Some results have been reported when used in combination therapy and particularly with immunotherapy, with some clinical trials still ongoing[192].
Subsequently discovered family- or member-specific inhibitors may offer more effective options as they might reduce toxicity and side effects observed with the first generation of non-selective HDACi. These include entinostat/MS-275 and NKL54, which favor class I enzymes other than HDAC8, and chidamide/CS055, which has been approved by the China Food and Drug Administration for peripheral T-cell lymphoma and advanced breast cancer[189]. The future perspective for HDACi lies in the identification of new isoform-selective and more effective inhibitors. These new agents could intervene more specifically to overcome cancer-related drug resistance, where the specific HDAC isoform determines the resistance phenotype. Innovative approaches such as proteolysis targeting chimera (PROTAC) also need to be utilized, although delivery of PROTAC-derived molecules to cancer tissue (due to large molecular mass and polarity) is an important limitation[193]. Less explored approaches such as miRNA-based therapies that mimic the various natural mRNAs that target these HDACs[22,194-198] or the development of small compounds that act as allosteric drugs to modify the assembly of the multiprotein complexes containing HDACs deserve special investigation.
Gene silencing occurs through the coordinated activities of various epigenetic regulators acting on different histone PTMs and DNA methylation. Therefore, concomitant treatment with inhibitors targeting other epigenetic regulators that may act synergistically with HDACs to suppress gene expression should be considered, especially if the resistant phenotype is due to epigenetic dysregulation. For example, DNMT inhibitors may synergize strongly with HDACi and activate gene expression differently than single-agent treatments[199-202]. Of particular interest is the recent development of dual DNMT1/HDAC inhibitors, which may have a similar synergistic effect to combined treatment with the two inhibitors, but with less toxicity[203].
CONCLUSION
The contribution of HDACs to drug resistance, particularly the zinc-dependent HDACs forming multiprotein complexes discussed in this review, has been investigated in different tumors and under different treatments. Over the last decade, evidence has accumulated for the contribution of HDACs to drug resistance in cancer. In general, their role in promoting resistance is predominant [Tables 1 and 2]. This has opened up the possibility of reversing the resistant phenotype with HDACi. In some studies, non-selective HDACi such as vorinostat/SAHA have been used and their effect could not be focused on the specific alteration. More recently, family-specific inhibitors have entered the market, potentially offering a more efficient means of intervention.
Summary of the contribution of class I HDACs (HDAC1/2/3) to drug resistance or (required) for drug action in the specified cancer type
HDAC | Drug | Cancer type | Contribution | Ref. |
HDAC1 | Cisplatin | Breast cancer | Required | [36] |
Cisplatin | Laryngeal cancer | Resistance | [39] | |
Cisplatin | NSCLC | Resistance | [40] | |
BTZ | MM | Resistance | [58] | |
5-FU | CRC | Required | [79] | |
PTX | NSCLC | Resistance | [91] | |
DTX | LAD | Resistance | [99,100] | |
ADT | Prostate cancer | Resistance | [142] | |
Oxaliplatin | Rectal cancer | Required | [172] | |
Olaparib | Ovarian cancer | Required | [181] | |
HDAC2 | Cisplatin | Ovarian cancer | Required | [43] |
5-FU | CRC | Required | [79] | |
Tamoxifen | Breast cancer | Resistance | [129] | |
Sunitinib | CCRC | Resistance | [158] | |
Olaparib | Ovarian cancer | Required | [181] | |
HDAC3 | Cisplatin | Lung cancer | Required | [44] |
Doxorubicin | AML | Resistance | [65] | |
DTX | Maxillary cancer | Resistance | [101] | |
DTX | Prostate cancer | Required | [102] | |
Exemestane | Breast cancer | Resistance | [126] | |
ADT | Prostate cancer | Resistance | [142] | |
Trametinib | PDAC | Resistance | [149] | |
Trametinib | NSCLC | Resistance | [153] |
Summary of the contribution of class IIa HDACs to drug resistance or drug action (required) in the specified cancer(s)
HDAC | Drug | Cancer type | Contribution | Ref. |
HDAC4 | Cisplatin | Ovarian cancer | Resistance | [45] |
Cisplatin | Gastric cancer | Resistance | [46] | |
Doxorubicin | Lung cancer | Resistance | [69] | |
Methotrexate | Osteosarcoma and CRC | Required | [82] | |
5-FU | Osteosarcoma | Required | [82] | |
5-FU | CRC | Resistance | [83] | |
Gemcitabine | PDAC | Resistance | [84] | |
PTX | PDAC | Resistance | [84] | |
DTX | Lung cancer | Resistance | [100] | |
Tamoxifen | Breast cancer | Required | [136] | |
Sorafenib | HCC | Resistance | [166] | |
PARPi | Breast and ovarian cancer | Required | [178] | |
HDAC5 | Cisplatin | Ovarian cancer | Resistance | [53] |
Tamoxifen | Breast cancer | Resistance | [129] | |
ADT | Prostate cancer | Resistance | [144] | |
Palbociclib | Prostate cancer | Required | [173] | |
PARPi | Breast and ovarian cancer | Required | [178] | |
HDAC7 | 5-FU | Lung cancer | Resistance | [81] |
Gemcitabine | PDAC | Resistance | [84] | |
PTX | PDAC | Resistance | [84] | |
HDAC9 | Tamoxifen | Breast cancer | Resistance | [135] |
MITR | PTX | Breast cancer | Resistance | [104] |
Frequently, alterations in HDACs associated with drug resistance occur (and have been studied) in the context of highly mutated cancer cell lines. These cells have also accumulated alterations in other key genes associated with drug resistance, including elements of the apoptotic machinery, the DDR, or elements controlling drug metabolism and availability. For these reasons, a combination therapy targeting some of these altered cellular responses should be used.
There are many examples of the contribution of these HDACs to the failure of specific cancer therapies with genetically validated results, which are summarized in Figure 3. However, we have very little information about the specific complexes involved in the specific context. This is particularly critical for HDAC1/2, where different multiprotein complexes have been mapped and defined. The contribution of the different complexes characterized by the deacetylase activities of class I HDACs could explain the large heterogeneity of mechanisms described in the literature under the supervision of these HDACs (class I and IIa). We have more differences than confirmations. Although the contribution of HDACs to resistance predominates, there are also cases where they are essential for treatment efficacy [Tables 1 and 2]. These contrasting results should not be a surprise. The context of specific cancer-associated changes and (again) the assembly of HDACs in different multiprotein complexes, whose composition may depend on cancer, are logical hypotheses to explain such differences.
Figure 3. Summary of the different cellular processes regulated by HDACs (1/2/3/4/5/7/9) that may determine anticancer drug resistance. Dysregulation of transcription can also affect the other biological processes mentioned. The different treatment options are outlined. HDACs: Histone deacetylases.
Although HDACs are important epigenetic regulators and important examples of changes in histone acetylation status associated with therapy resistance have been documented, there are other cases involving the regulation of non-histone proteins[45,49,50,53,65,81,84,130]. Certainly, their contribution as epigenetic regulators is underestimated. In particular, their role in shaping H3K27ac at enhancers and super-enhancers and how this contributes to drug resistance is poorly studied[204-211]. An obvious aspect that we should also consider is the relationship with the antagonizing enzymes, the KATs, both in terms of contribution to drug resistance and possible targets to improve therapy depending on the specific environment.
The ultimate goal of these studies should be to gain insight into the contribution of HDAC-dependent changes in a patient-specific manner and thus stratify patients to optimize therapeutic strategy. This would optimize benefits for patients and costs for healthcare systems. Of course, the question is not easy to answer, as the nature (point mutations, changes in expression, different PTMs, assembly into different multiprotein complexes, etc.) and the number of alterations can vary greatly. However, since these HDACs are primarily epigenetic regulators, a defined signature of changes in gene expression could be a possible indirect method for unmasking their alterations.
DECLARATIONS
Authors’ contributions
Design of the review, performed literature search and interpretation, and wrote the manuscript: Minisini M, Mascaro M
Made substantial contributions to the conception and design of the review, performed literature search and interpretation, generated the figures, supervised, and wrote the manuscript: Brancolini C
Availability of data and materials
Not applicable.
Financial support and sponsorship
Research work in the CB lab is supported by AIRC under IG 2021 - ID. 26200 project; Interreg Italia-Osterreich ITAT11-018 SENECA, CIB “Modelli innovativi per applicazioni di scienze omiche avanzate - Tecnologie innovative per le scienze omiche avanzate in modelli umani e vegetali”.
Conflicts of interest
All authors declared that there are no conflicts of interest.
Ethical approval and consent to participate
Not applicable.
Consent for publication
Not applicable.
Copyright
© The Author(s) 2024.
REFERENCES
1. World Bank Group. Metadata glossary. Cause of death, by injury (% of total). Available from: https://databank.worldbank.org/metadataglossary/world-development-indicators/series/SH.DTH.INJR.ZS. [Last accessed on 20 Nov 2024]
2. Sung H, Ferlay J, Siegel RL, et al. Global cancer statistics 2020: GLOBOCAN estimates of incidence and mortality worldwide for 36 cancers in 185 countries. CA Cancer J Clin 2021;71:209-49.
3. Collaborative and National Institute for Health Research Global Health Research Unit on Global Surgery. Global variation in postoperative mortality and complications after cancer surgery: a multicentre, prospective cohort study in 82 countries. Lancet 2021;397:387-97.
4. Mansoori B, Mohammadi A, Davudian S, Shirjang S, Baradaran B. The different mechanisms of cancer drug resistance: a brief review. Adv Pharm Bull 2017;7:339-48.
5. Mollaei M, Hassan ZM, Khorshidi F, Langroudi L. Chemotherapeutic drugs: cell death- and resistance-related signaling pathways. Are they really as smart as the tumor cells? Transl Oncol 2021;14:101056.
6. Luria SE, Delbrück M. Mutations of bacteria from virus sensitivity to virus resistance. Genetics 1943;28:491-511.
7. Goldie JH, Coldman AJ. A mathematic model for relating the drug sensitivity of tumors to their spontaneous mutation rate. Cancer Treat Rep 1979;63:1727-33.
9. Brancolini C, Gagliano T, Minisini M. HDACs and the epigenetic plasticity of cancer cells: target the complexity. Pharmacol Ther 2022;238:108190.
10. Bahl S, Seto E. Regulation of histone deacetylase activities and functions by phosphorylation and its physiological relevance. Cell Mol Life Sci 2021;78:427-45.
11. Kelly RDW, Chandru A, Watson PJ, et al. Histone deacetylase (HDAC) 1 and 2 complexes regulate both histone acetylation and crotonylation in vivo. Sci Rep 2018;8:14690.
12. Zhao Y, Garcia BA. Comprehensive catalog of currently documented histone modifications. Cold Spring Harb Perspect Biol 2015;7:a025064.
13. Porter NJ, Christianson DW. Structure, mechanism, and inhibition of the zinc-dependent histone deacetylases. Curr Opin Struct Biol 2019;59:9-18.
14. Bantscheff M, Hopf C, Savitski MM, et al. Chemoproteomics profiling of HDAC inhibitors reveals selective targeting of HDAC complexes. Nat Biotechnol 2011;29:255-65.
15. Millard CJ, Watson PJ, Fairall L, Schwabe JWR. Targeting class I histone deacetylases in a “complex” environment. Trends Pharmacol Sci 2017;38:363-77.
16. BioGRID4.4. Available from: https://thebiogrid.org. [Last accessed on 18 Nov 2024].
17. Di Giorgio E, Hancock WW, Brancolini C. MEF2 and the tumorigenic process, hic sunt leones. Biochim Biophys Acta Rev Cancer 2018;1870:261-73.
18. Di Giorgio E, Dalla E, Tolotto V, et al. HDAC4 influences the DNA damage response and counteracts senescence by assembling with HDAC1/HDAC2 to control H2BK120 acetylation and homology-directed repair. Nucleic Acids Res 2024;52:8218-40.
19. Park SY, Kim GS, Hwang HJ, et al. Structural basis of the specific interaction of SMRT corepressor with histone deacetylase 4. Nucleic Acids Res 2018;46:11776-88.
20. Lahm A, Paolini C, Pallaoro M, et al. Unraveling the hidden catalytic activity of vertebrate class IIa histone deacetylases. Proc Natl Acad Sci U S A 2007;104:17335-40.
21. Zhang Y, Andrade R, Hanna AA, Pflum MKH. Evidence that HDAC7 acts as an epigenetic “reader” of AR acetylation through NCoR-HDAC3 dissociation. Cell Chem Biol 2022;29:1162-73.e5.
22. Di Giorgio E, Brancolini C. Regulation of class IIa HDAC activities: it is not only matter of subcellular localization. Epigenomics 2016;8:251-69.
23. Dai S, Guo L, Dey R, et al. Structural insights into the HDAC4-MEF2A-DNA complex and its implication in long-range transcriptional regulation. Nucleic Acids Res 2024;52:2711-23.
24. Biersack B, Nitzsche B, Höpfner M. Immunomodulatory properties of HDAC6 inhibitors in cancer diseases: new chances for sophisticated drug design and treatment optimization. Semin Cell Dev Biol 2024;154:286-94.
25. Pulya S, Amin SA, Adhikari N, Biswas S, Jha T, Ghosh B. HDAC6 as privileged target in drug discovery: a perspective. Pharmacol Res 2021;163:105274.
26. Lambona C, Zwergel C, Fioravanti R, Valente S, Mai A. Histone deacetylase 10: a polyamine deacetylase from the crystal structure to the first inhibitors. Curr Opin Struct Biol 2023;82:102668.
27. Hai Y, Shinsky SA, Porter NJ, Christianson DW. Histone deacetylase 10 structure and molecular function as a polyamine deacetylase. Nat Commun 2017;8:15368.
29. Milazzo G, Mercatelli D, Di Muzio G, et al. Histone deacetylases (HDACs): evolution, specificity, role in transcriptional complexes, and pharmacological actionability. Genes 2020;11:E556.
30. Li G, Tian Y, Zhu WG. The roles of histone deacetylases and their inhibitors in cancer therapy. Front Cell Dev Biol 2020;8:576946.
31. Emmett MJ, Lazar MA. Integrative regulation of physiology by histone deacetylase 3. Nat Rev Mol Cell Biol 2019;20:102-15.
33. Song M, Cui M, Liu K. Therapeutic strategies to overcome cisplatin resistance in ovarian cancer. Eur J Med Chem 2022;232:114205.
34. Spears N, Lopes F, Stefansdottir A, et al. Ovarian damage from chemotherapy and current approaches to its protection. Hum Reprod Update 2019;25:673-93.
35. Galluzzi L, Senovilla L, Vitale I, et al. Molecular mechanisms of cisplatin resistance. Oncogene 2012;31:1869-83.
36. Zhang Z, Liu X, Li L, et al. SNP rs4971059 predisposes to breast carcinogenesis and chemoresistance via TRIM46-mediated HDAC1 degradation. EMBO J 2021;40:e107974.
37. Mylavarapu S, Das A, Roy M. Role of BRCA mutations in the modulation of response to platinum therapy. Front Oncol 2018;8:16.
38. Zhu Y, Liu Y, Zhang C, et al. Tamoxifen-resistant breast cancer cells are resistant to DNA-damaging chemotherapy because of upregulated BARD1 and BRCA1. Nat Commun 2018;9:1595.
39. Ding S, Tang Z, Jiang Y, et al. HDAC1 regulates the chemosensitivity of laryngeal carcinoma cells via modulation of interleukin-8 expression. Eur J Pharmacol 2021;896:173923.
40. Sun Y, Bao X, Ren Y, et al. Targeting HDAC/OAZ1 axis with a novel inhibitor effectively reverses cisplatin resistance in non-small cell lung cancer. Cell Death Dis 2019;10:400.
41. Kurian L, Palanimurugan R, Gödderz D, Dohmen RJ. Polyamine sensing by nascent ornithine decarboxylase antizyme stimulates decoding of its mRNA. Nature 2011;477:490-4.
42. Sun JM, Chen HY, Davie JR. Differential distribution of unmodified and phosphorylated histone deacetylase 2 in chromatin. J Biol Chem 2007;282:33227-36.
43. Huang R, Langdon SP, Tse M, et al. The role of HDAC2 in chromatin remodelling and response to chemotherapy in ovarian cancer. Oncotarget 2016;7:4695-711.
44. Wang H, Fu C, Du J, et al. Enhanced histone H3 acetylation of the PD-L1 promoter via the COP1/c-Jun/HDAC3 axis is required for PD-L1 expression in drug-resistant cancer cells. J Exp Clin Cancer Res 2020;39:29.
45. Stronach EA, Alfraidi A, Rama N, et al. HDAC4-regulated STAT1 activation mediates platinum resistance in ovarian cancer. Cancer Res 2011;71:4412-22.
46. Spaety ME, Gries A, Badie A, et al. HDAC4 levels control sensibility toward cisplatin in gastric cancer via the p53-p73/BIK pathway. Cancers 2019;11:1747.
47. Brachova P, Thiel KW, Leslie KK. The consequence of oncomorphic TP53 mutations in ovarian cancer. Int J Mol Sci 2013;14:19257-75.
48. Reles A, Wen WH, Schmider A, et al. Correlation of p53 mutations with resistance to platinum-based chemotherapy and shortened survival in ovarian cancer. Clin Cancer Res 2001;7:2984-97.
49. Zhang X, Qi Z, Yin H, Yang G. Interaction between p53 and Ras signaling controls cisplatin resistance via HDAC4- and HIF-1α-mediated regulation of apoptosis and autophagy. Theranostics 2019;9:1096-114.
50. Minisini M, Cricchi E, Brancolini C. Acetylation and phosphorylation in the regulation of hypoxia-inducible factor activities: additional options to modulate adaptations to changes in oxygen levels. Life 2023;14:20.
51. Aydin B, Arga KY. Co-expression network analysis elucidated a core module in association with prognosis of non-functioning non-invasive human pituitary adenoma. Front Endocrinol 2019;10:361.
52. Guo C, Tang Y, Zhang Y, Li G. Mining TCGA data for key biomarkers related to immune microenvironment in endometrial cancer by immune score and weighted correlation network analysis. Front Mol Biosci 2021;8:645388.
53. Zhang Y, Qiu JG, Jia XY, et al. METTL3-mediated N6-methyladenosine modification and HDAC5/YY1 promote IFFO1 downregulation in tumor development and chemo-resistance. Cancer Lett 2023;553:215971.
54. Sucharov CC, Langer S, Bristow M, Leinwand L. Shuttling of HDAC5 in H9C2 cells regulates YY1 function through CaMKIV/PKD and PP2A. Am J Physiol Cell Physiol 2006;291:C1029-37.
55. Sucharov CC, Dockstader K, McKinsey TA. YY1 protects cardiac myocytes from pathologic hypertrophy by interacting with HDAC5. Mol Biol Cell 2008;19:4141-53.
56. Qian S, Wang W, Li M. Transcriptional factor Yin Yang 1 facilitates the stemness of ovarian cancer via suppressing miR-99a activity through enhancing its deacetylation level. Biomed Pharmacother 2020;126:110085.
57. Zhou L, Xu X, Liu H, et al. Prognosis analysis of histone deacetylases mRNA expression in ovarian cancer patients. J Cancer 2018;9:4547-55.
58. Li X, Wang S, Xie Y, et al. Deacetylation induced nuclear condensation of HP1γ promotes multiple myeloma drug resistance. Nat Commun 2023;14:1290.
59. Kumar A, Kono H. Heterochromatin protein 1 (HP1): interactions with itself and chromatin components. Biophys Rev 2020;12:387-400.
60. Zeng W, Ball AR Jr, Yokomori K. HP1: heterochromatin binding proteins working the genome. Epigenetics 2010;5:287-92.
61. Casale AM, Cappucci U, Piacentini L. Unravelling HP1 functions: post-transcriptional regulation of stem cell fate. Chromosoma 2021;130:103-11.
62. Arcamone F, Cassinelli G, Fantini G, et al. Adriamycin, 14-hydroxydaunomycin, a new antitumor antibiotic from S. peucetius var. caesius. Biotechnol Bioeng 1969;11:1101-10.
63. Cox J, Weinman S. Mechanisms of doxorubicin resistance in hepatocellular carcinoma. Hepat Oncol 2016;3:57-9.
64. Kim SJ, Kim HS, Seo YR. Understanding of ROS-inducing strategy in anticancer therapy. Oxid Med Cell Longev 2019;2019:5381692.
65. Long J, Fang WY, Chang L, et al. Targeting HDAC3, a new partner protein of AKT in the reversal of chemoresistance in acute myeloid leukemia via DNA damage response. Leukemia 2017;31:2761-70.
66. Grandage VL, Gale RE, Linch DC, Khwaja A. PI3-kinase/Akt is constitutively active in primary acute myeloid leukaemia cells and regulates survival and chemoresistance via NF-kappaB, mapkinase and p53 pathways. Leukemia 2005;19:586-94.
67. West KA, Castillo SS, Dennis PA. Activation of the PI3K/Akt pathway and chemotherapeutic resistance. Drug Resist Updat 2002;5:234-48.
68. Fryknäs M, Dhar S, Oberg F, et al. STAT1 signaling is associated with acquired crossresistance to doxorubicin and radiation in myeloma cell lines. Int J Cancer 2007;120:189-95.
69. Kaowinn S, Jun SW, Kim CS, et al. Increased EGFR expression induced by a novel oncogene, CUG2, confers resistance to doxorubicin through Stat1-HDAC4 signaling. Cell Oncol 2017;40:549-61.
70. Drury AN, Szent-Györgyi A. The physiological activity of adenine compounds with especial reference to their action upon the mammalian heart. J Physiol 1929;68:213-37.
71. Aumer T, Gremmelmaier CB, Runtsch LS, et al. Comprehensive comparison between azacytidine and decitabine treatment in an acute myeloid leukemia cell line. Clin Epigenetics 2022;14:113.
72. Wu HL, Gong Y, Ji P, Xie YF, Jiang YZ, Liu GY. Targeting nucleotide metabolism: a promising approach to enhance cancer immunotherapy. J Hematol Oncol 2022;15:45.
73. Beutel AK, Halbrook CJ. Barriers and opportunities for gemcitabine in pancreatic cancer therapy. Am J Physiol Cell Physiol 2023;324:C540-52.
74. Meng XN, Ma JF, Liu YH, et al. Dynamic genomic changes in methotrexate-resistant human cancer cell lines beyond DHFR amplification suggest potential new targets for preventing drug resistance. Br J Cancer 2024;130:1819-27.
75. Alnuqaydan AM, Rah B, Almutary AG, Chauhan SS. Synergistic antitumor effect of 5-fluorouracil and withaferin-A induces endoplasmic reticulum stress-mediated autophagy and apoptosis in colorectal cancer cells. Am J Cancer Res 2020;10:799-815.
76. Wang M, Han D, Yuan Z, et al. Long non-coding RNA H19 confers 5-Fu resistance in colorectal cancer by promoting SIRT1-mediated autophagy. Cell Death Dis 2018;9:1149.
77. Offer SM, Wegner NJ, Fossum C, Wang K, Diasio RB. Phenotypic profiling of DPYD variations relevant to 5-fluorouracil sensitivity using real-time cellular analysis and in vitro measurement of enzyme activity. Cancer Res 2013;73:1958-68.
78. Hait NC, Allegood J, Maceyka M, et al. Regulation of histone acetylation in the nucleus by sphingosine-1-phosphate. Science 2009;325:1254-7.
79. Zhang YH, Shi WN, Wu SH, et al. SphK2 confers 5-fluorouracil resistance to colorectal cancer via upregulating H3K56ac-mediated DPD expression. Oncogene 2020;39:5214-27.
80. Kiweler N, Schwarz H, Nguyen A, et al. The epigenetic modifier HDAC2 and the checkpoint kinase ATM determine the responses of microsatellite instable colorectal cancer cells to 5-fluorouracil. Cell Biol Toxicol 2023;39:2401-19.
81. Wen W, Ding J, Sun W, et al. Suppression of cyclin D1 by hypoxia-inducible factor-1 via direct mechanism inhibits the proliferation and 5-fluorouracil-induced apoptosis of A549 cells. Cancer Res 2010;70:2010-9.
82. Song B, Wang Y, Xi Y, et al. Mechanism of chemoresistance mediated by miR-140 in human osteosarcoma and colon cancer cells. Oncogene 2009;28:4065-74.
83. Zhao L, Chen H, Zhang Q, Ma J, Hu H, Xu L. ATF4-mediated microRNA-145/HDAC4/p53 axis affects resistance of colorectal cancer cells to 5-fluorouracil by regulating autophagy. Cancer Chemother Pharmacol 2022;89:595-607.
84. Zeng Y, Yin L, Zhou J, et al. MARK2 regulates chemotherapeutic responses through class IIa HDAC-YAP axis in pancreatic cancer. Oncogene 2022;41:3859-75.
85. Zhao S, Tang Y, Wang R, Najafi M. Mechanisms of cancer cell death induction by paclitaxel: an updated review. Apoptosis 2022;27:647-67.
86. Snyder JP, Nettles JH, Cornett B, Downing KH, Nogales E. The binding conformation of Taxol in beta-tubulin: a model based on electron crystallographic density. Proc Natl Acad Sci U S A 2001;98:5312-6.
87. Mosca L, Ilari A, Fazi F, Assaraf YG, Colotti G. Taxanes in cancer treatment: activity, chemoresistance and its overcoming. Drug Resist Updat 2021;54:100742.
88. Ismail U, Killeen RB. Taxane toxicity. In: StatPearls. 2023. Available from: https://www.ncbi.nlm.nih.gov/books/NBK589655/. [Last accessed on 18 Nov 2024].
89. Holohan C, Van Schaeybroeck S, Longley DB, Johnston PG. Cancer drug resistance: an evolving paradigm. Nat Rev Cancer 2013;13:714-26.
90. Liao D, Zhang W, Gupta P, et al. Tetrandrine interaction with ABCB1 reverses multidrug resistance in cancer cells through competition with anti-cancer drugs followed by downregulation of ABCB1 expression. Molecules 2019;24:4383.
91. Wang L, Li H, Ren Y, et al. Targeting HDAC with a novel inhibitor effectively reverses paclitaxel resistance in non-small cell lung cancer via multiple mechanisms. Cell Death Dis 2016;7:e2063.
92. Kadamb R, Mittal S, Bansal N, Batra H, Saluja D. Sin3: insight into its transcription regulatory functions. Eur J Cell Biol 2013;92:237-46.
93. Merle NS, Church SE, Fremeaux-Bacchi V, Roumenina LT. Complement system part I - molecular mechanisms of activation and regulation. Front Immunol 2015;6:262.
94. Merle NS, Noe R, Halbwachs-Mecarelli L, Fremeaux-Bacchi V, Roumenina LT. Complement system part II: role in immunity. Front Immunol 2015;6:257.
95. Hollander MC, Fornace AJ Jr. Genomic instability, centrosome amplification, cell cycle checkpoints and Gadd45a. Oncogene 2002;21:6228-33.
96. Wang X, Hao Y, Chen J, et al. Nuclear complement C3b promotes paclitaxel resistance by assembling the SIN3A/HDAC1/2 complex in non-small cell lung cancer. Cell Death Dis 2023;14:351.
97. Broux M, Prieto C, Demeyer S, et al. Suz12 inactivation cooperates with JAK3 mutant signaling in the development of T-cell acute lymphoblastic leukemia. Blood 2019;134:1323-36.
98. Conway E, Healy E, Bracken AP. PRC2 mediated H3K27 methylations in cellular identity and cancer. Curr Opin Cell Biol 2015;37:42-8.
99. Jiang M, Qi F, Zhang K, et al. MARCKSL1-2 reverses docetaxel-resistance of lung adenocarcinoma cells by recruiting SUZ12 to suppress HDAC1 and elevate miR-200b. Mol Cancer 2022;21:150.
100. Chen DQ, Pan BZ, Huang JY, et al. HDAC 1/4-mediated silencing of microRNA-200b promotes chemoresistance in human lung adenocarcinoma cells. Oncotarget 2014;5:3333-49.
101. Narita N, Fujieda S, Kimura Y, et al. Suppression of histone deacetylase 3 (HDAC3) enhances apoptosis induced by paclitaxel in human maxillary cancer cells in vitro and in vivo. Biochem Biophys Res Commun 2010;396:310-6.
102. Jia J, Zhang HB, Shi Q, et al. Erratum: KLF5 downregulation desensitizes castration-resistant prostate cancer cells to docetaxel by increasing BECN1 expression and inducing cell autophagy: Erratum. Theranostics 2023;13:2962-3.
103. Brancolini C, Di Giorgio E, Formisano L, Gagliano T. Quis custodiet ipsos custodes (who controls the controllers)? Two decades of studies on HDAC9. Life 2021;11:90.
104. Lian B, Pei YC, Jiang YZ, et al. Truncated HDAC9 identified by integrated genome-wide screen as the key modulator for paclitaxel resistance in triple-negative breast cancer. Theranostics 2020;10:11092-109.
105. Chudobová J, Zempel H. Microtubule affinity regulating kinase (MARK/Par1) isoforms differentially regulate Alzheimer-like TAU missorting and Aβ-mediated synapse pathology. Neural Regen Res 2023;18:335-6.
106. Natalia MA, Alejandro GT, Virginia TJ, Alvarez-Salas LM. MARK1 is a novel target for miR-125a-5p: implications for cell migration in cervical tumor cells. Microrna 2018;7:54-61.
107. Miki Y. Hormone-dependent cancers: new aspects on biochemistry and molecular pathology. Int J Mol Sci 2023;24:10830.
108. Mouridsen H, Palshof T, Patterson J, Battersby L. Tamoxifen in advanced breast cancer. Cancer Treat Rev 1978;5:131-41.
109. Kowalczyk W, Waliszczak G, Jach R, Dulińska-Litewka J. Steroid receptors in breast cancer: understanding of molecular function as a basis for effective therapy development. Cancers 2021;13:4779.
110. Murphy CG, Dickler MN. Endocrine resistance in hormone-responsive breast cancer: mechanisms and therapeutic strategies. Endocr Relat Cancer 2016;23:R337-52.
111. Achinger-Kawecka J, Valdes-Mora F, Luu PL, et al. Epigenetic reprogramming at estrogen-receptor binding sites alters 3D chromatin landscape in endocrine-resistant breast cancer. Nat Commun 2020;11:320.
112. Davis T, Kennedy C, Chiew YE, Clarke CL, deFazio A. Histone deacetylase inhibitors decrease proliferation and modulate cell cycle gene expression in normal mammary epithelial cells. Clin Cancer Res 2000;6:4334-42.
113. Vigushin DM, Ali S, Pace PE, et al. Trichostatin A is a histone deacetylase inhibitor with potent antitumor activity against breast cancer in vivo. Clin Cancer Res 2001;7:971-6.
114. Margueron R, Licznar A, Lazennec G, Vignon F, Cavaillès V. Oestrogen receptor alpha increases p21(WAF1/CIP1) gene expression and the antiproliferative activity of histone deacetylase inhibitors in human breast cancer cells. J Endocrinol 2003;179:41-53.
115. Alao JP, Stavropoulou AV, Lam EW, Coombes RC, Vigushin DM. Histone deacetylase inhibitor, trichostatin A induces ubiquitin-dependent cyclin D1 degradation in MCF-7 breast cancer cells. Mol Cancer 2006;5:8.
116. Connolly RM, Zhao F, Miller KD, et al. E2112: randomized phase III trial of endocrine therapy plus entinostat or placebo in hormone receptor-positive advanced breast cancer. A trial of the ECOG-ACRIN Cancer Research Group. J Clin Oncol 2021;39:3171-81.
117. Macaluso M, Cinti C, Russo G, Russo A, Giordano A. pRb2/p130-E2F4/5-HDAC1-SUV39H1-p300 and pRb2/p130-E2F4/5-HDAC1-SUV39H1-DNMT1 multimolecular complexes mediate the transcription of estrogen receptor-alpha in breast cancer. Oncogene 2003;22:3511-7.
118. Liu XF, Bagchi MK. Recruitment of distinct chromatin-modifying complexes by tamoxifen-complexed estrogen receptor at natural target gene promoters in vivo. J Biol Chem 2004;279:15050-8.
119. Demirpence E, Semlali A, Oliva J, et al. An estrogen-responsive element-targeted histone deacetylase enzyme has an antiestrogen activity that differs from that of hydroxytamoxifen. Cancer Res 2002;62:6519-28.
120. Konduri SD, Medisetty R, Liu W, et al. Mechanisms of estrogen receptor antagonism toward p53 and its implications in breast cancer therapeutic response and stem cell regulation. Proc Natl Acad Sci U S A 2010;107:15081-6.
121. Suzuki A, Sanda N, Miyawaki Y, et al. Down-regulation of PROS1 gene expression by 17beta-estradiol via estrogen receptor alpha (ERalpha)-Sp1 interaction recruiting receptor-interacting protein 140 and the corepressor-HDAC3 complex. J Biol Chem 2010;285:13444-53.
122. Leong H, Sloan JR, Nash PD, Greene GL. Recruitment of histone deacetylase 4 to the N-terminal region of estrogen receptor alpha. Mol Endocrinol 2005;19:2930-42.
123. Duong V, Bret C, Altucci L, et al. Specific activity of class II histone deacetylases in human breast cancer cells. Mol Cancer Res 2008;6:1908-19.
124. Malik S, Jiang S, Garee JP, et al. Histone deacetylase 7 and FoxA1 in estrogen-mediated repression of RPRM. Mol Cell Biol 2010;30:399-412.
125. Kurtev V, Margueron R, Kroboth K, Ogris E, Cavailles V, Seiser C. Transcriptional regulation by the repressor of estrogen receptor activity via recruitment of histone deacetylases. J Biol Chem 2004;279:24834-43.
126. Zhou J, Zhu M, Wang Q, et al. SERPINA3-ANKRD11-HDAC3 pathway induced aromatase inhibitor resistance in breast cancer can be reversed by HDAC3 inhibition. Commun Biol 2023;6:695.
127. Yang F, Ma Q, Liu Z, et al. Glucocorticoid receptor:MegaTrans switching mediates the repression of an ERα-regulated transcriptional program. Mol Cell 2017;66:321-31.e6.
128. Emmett MJ, Lim HW, Jager J, et al. Histone deacetylase 3 prepares brown adipose tissue for acute thermogenic challenge. Nature 2017;546:544-8.
129. Huang WT, Tsai YH, Chen SH, et al. HDAC2 and HDAC5 up-regulations modulate survivin and miR-125a-5p expressions and promote hormone therapy resistance in estrogen receptor positive breast cancer cells. Front Pharmacol 2017;8:902.
130. Xue Y, Lian W, Zhi J, et al. HDAC5-mediated deacetylation and nuclear localisation of SOX9 is critical for tamoxifen resistance in breast cancer. Br J Cancer 2019;121:1039-49.
131. Choi SR, Hwang CY, Lee J, Cho KH. Network analysis identifies regulators of basal-like breast cancer reprogramming and endocrine therapy vulnerability. Cancer Res 2022;82:320-33.
132. Liu C, Liu L, Chen X, et al. Sox9 regulates self-renewal and tumorigenicity by promoting symmetrical cell division of cancer stem cells in hepatocellular carcinoma. Hepatology 2016;64:117-29.
133. Jeselsohn R, Cornwell M, Pun M, et al. Embryonic transcription factor SOX9 drives breast cancer endocrine resistance. Proc Natl Acad Sci U S A 2017;114:E4482-91.
134. Jin K, Park S, Teo WW, et al. HOXB7 is an ERα cofactor in the activation of HER2 and multiple ER target genes leading to endocrine resistance. Cancer Discov 2015;5:944-59.
135. Linares A, Assou S, Lapierre M, et al. Increased expression of the HDAC9 gene is associated with antiestrogen resistance of breast cancers. Mol Oncol 2019;13:1534-47.
136. Ahmad A, Ginnebaugh KR, Yin S, Bollig-Fischer A, Reddy KB, Sarkar FH. Functional role of miR-10b in tamoxifen resistance of ER-positive breast cancer cells through down-regulation of HDAC4. BMC Cancer 2015;15:540.
137. Katsogiannou M, Ziouziou H, Karaki S, Andrieu C, Henry de Villeneuve M, Rocchi P. The hallmarks of castration-resistant prostate cancers. Cancer Treat Rev 2015;41:588-97.
138. Westaby D, Fenor de La Maza MLD, Paschalis A, et al. A new old target: androgen receptor signaling and advanced prostate cancer. Annu Rev Pharmacol Toxicol 2022;62:131-53.
139. Biersack B, Nitzsche B, Höpfner M. HDAC inhibitors with potential to overcome drug resistance in castration-resistant prostate cancer. Cancer Drug Resist 2022;5:64-79.
140. Welsbie DS, Xu J, Chen Y, et al. Histone deacetylases are required for androgen receptor function in hormone-sensitive and castrate-resistant prostate cancer. Cancer Res 2009;69:958-66.
141. McLeod AB, Stice JP, Wardell SE, Alley HM, Chang CY, McDonnell DP. Validation of histone deacetylase 3 as a therapeutic target in castration-resistant prostate cancer. Prostate 2018;78:266-77.
142. Jia L, Wu D, Wang Y, et al. Orphan nuclear receptor TLX contributes to androgen insensitivity in castration-resistant prostate cancer via its repression of androgen receptor transcription. Oncogene 2018;37:3340-55.
143. Liu P, Li S, Gan L, Kao TP, Huang H. A transcription-independent function of FOXO1 in inhibition of androgen-independent activation of the androgen receptor in prostate cancer cells. Cancer Res 2008;68:10290-9.
144. Li X, Wang Y, Deng S, et al. Loss of SYNCRIP unleashes APOBEC-driven mutagenesis, tumor heterogeneity, and AR-targeted therapy resistance in prostate cancer. Cancer Cell 2023;41:1427-49.e12.
145. Yang Y, Tse AK, Li P, et al. Inhibition of androgen receptor activity by histone deacetylase 4 through receptor SUMOylation. Oncogene 2011;30:2207-18.
146. Degirmenci U, Yap J, Sim YRM, Qin S, Hu J. Drug resistance in targeted cancer therapies with RAF inhibitors. Cancer Drug Resist 2021;4:665-83.
147. Rinne N, Christie EL, Ardasheva A, et al. Targeting the PI3K/AKT/mTOR pathway in epithelial ovarian cancer, therapeutic treatment options for platinum-resistant ovarian cancer. Cancer Drug Resist 2021;4:573-95.
148. Zielli T, Labidi-Galy I, Del Grande M, Sessa C, Colombo I. The clinical challenges of homologous recombination proficiency in ovarian cancer: from intrinsic resistance to new treatment opportunities. Cancer Drug Resist 2023;6:499-516.
149. Wang Z, Hausmann S, Lyu R, et al. SETD5-coordinated chromatin reprogramming regulates adaptive resistance to targeted pancreatic cancer therapy. Cancer Cell 2020;37:834-49.e13.
150. Martinelli E, Morgillo F, Troiani T, Ciardiello F. Cancer resistance to therapies against the EGFR-RAS-RAF pathway: the role of MEK. Cancer Treat Rev 2017;53:61-9.
151. Infante JR, Somer BG, Park JO, et al. A randomised, double-blind, placebo-controlled trial of trametinib, an oral MEK inhibitor, in combination with gemcitabine for patients with untreated metastatic adenocarcinoma of the pancreas. Eur J Cancer 2014;50:2072-81.
152. Sun Y, Daemen A, Hatzivassiliou G, et al. Metabolic and transcriptional profiling reveals pyruvate dehydrogenase kinase 4 as a mediator of epithelial-mesenchymal transition and drug resistance in tumor cells. Cancer Metab 2014;2:20.
153. Eichner LJ, Curtis SD, Brun SN, et al. HDAC3 is critical in tumor development and therapeutic resistance in Kras-mutant non-small cell lung cancer. Sci Adv 2023;9:eadd3243.
154. Iacovelli R, Albiges L, Escudier B. Emerging tyrosine kinase inhibitors for the treatment of renal cancer. Expert Opin Emerg Drugs 2015;20:379-92.
155. Makhov P, Joshi S, Ghatalia P, Kutikov A, Uzzo RG, Kolenko VM. Resistance to systemic therapies in clear cell renal cell carcinoma: mechanisms and management strategies. Mol Cancer Ther 2018;17:1355-64.
156. Xiong W, Zhang B, Yu H, Zhu L, Yi L, Jin X. RRM2 regulates sensitivity to sunitinib and PD-1 blockade in renal cancer by stabilizing ANXA1 and activating the AKT pathway. Adv Sci 2021;8:e2100881.
157. von Hagen F, Gundert L, Strick A, et al. N6-Methyladenosine (m6 A) readers are dysregulated in renal cell carcinoma. Mol Carcinog 2021;60:354-62.
158. Li W, Ye K, Li X, et al. YTHDC1 is downregulated by the YY1/HDAC2 complex and controls the sensitivity of ccRCC to sunitinib by targeting the ANXA1-MAPK pathway. J Exp Clin Cancer Res 2022;41:250.
159. Abdelgalil AA, Alkahtani HM, Al-Jenoobi FI. Chapter Four - Sorafenib. In: Profiles of drug substances, excipients, and related methodology. Elsevier; 2019. pp. 239-66.
160. Kudo M. A paradigm change in the treatment strategy for hepatocellular carcinoma. Liver Cancer 2020;9:367-77.
161. Tang W, Chen Z, Zhang W, et al. The mechanisms of sorafenib resistance in hepatocellular carcinoma: theoretical basis and therapeutic aspects. Signal Transduct Target Ther 2020;5:87.
162. Grégoire S, Tremblay AM, Xiao L, et al. Control of MEF2 transcriptional activity by coordinated phosphorylation and sumoylation. J Biol Chem 2006;281:4423-33.
163. Clocchiatti A, Di Giorgio E, Viviani G, et al. The MEF2-HDAC axis controls proliferation of mammary epithelial cells and acini formation in vitro. J Cell Sci 2015;128:3961-76.
164. Rudalska R, Dauch D, Longerich T, et al. In vivo RNAi screening identifies a mechanism of sorafenib resistance in liver cancer. Nat Med 2014;20:1138-46.
165. Xu Y, Huang J, Ma L, et al. MicroRNA-122 confers sorafenib resistance to hepatocellular carcinoma cells by targeting IGF-1R to regulate RAS/RAF/ERK signaling pathways. Cancer Lett 2016;371:171-81.
166. Ma Q, Xu Q, Zhao J, et al. Coupling HDAC4 with transcriptional factor MEF2D abrogates SPRY4-mediated suppression of ERK activation and elicits hepatocellular carcinoma drug resistance. Cancer Lett 2021;520:243-54.
167. Isaacs JT, Dalrymple SL, Antony L, et al. Third generation quinoline-3-carboxamide transcriptional disrupter of HDAC4, HIF-1α, and MEF-2 signaling for metastatic castration-resistant prostate cancer. Prostate 2023;83:1470-93.
168. Papadimitriou MC, Pazaiti A, Iliakopoulos K, Markouli M, Michalaki V, Papadimitriou CA. Resistance to CDK4/6 inhibition: mechanisms and strategies to overcome a therapeutic problem in the treatment of hormone receptor-positive metastatic breast cancer. Biochim Biophys Acta Mol Cell Res 2022;1869:119346.
169. Magge T, Rajendran S, Brufsky AM, Foldi J. CDK4/6 inhibitors: the devil is in the detail. Curr Oncol Rep 2024;26:665-78.
170. Luo RX, Postigo AA, Dean DC. Rb interacts with histone deacetylase to repress transcription. Cell 1998;92:463-73.
171. Sanidas I, Morris R, Fella KA, et al. A code of mono-phosphorylation modulates the function of RB. Mol Cell 2019;73:985-1000.e6.
172. Yu Z, Deng P, Chen Y, et al. Pharmacological modulation of RB1 activity mitigates resistance to neoadjuvant chemotherapy in locally advanced rectal cancer. Proc Natl Acad Sci U S A 2024;121:e2304619121.
173. Zhou Y, Jin X, Ma J, et al. HDAC5 loss impairs RB repression of pro-oncogenic genes and confers CDK4/6 inhibitor resistance in cancer. Cancer Res 2021;81:1486-99.
174. Marek L, Hamacher A, Hansen FK, et al. Histone deacetylase (HDAC) inhibitors with a novel connecting unit linker region reveal a selectivity profile for HDAC4 and HDAC5 with improved activity against chemoresistant cancer cells. J Med Chem 2013;56:427-36.
175. Di Giorgio E, Gagliostro E, Brancolini C. Selective class IIa HDAC inhibitors: myth or reality. Cell Mol Life Sci 2015;72:73-86.
176. Konstantinopoulos PA, Ceccaldi R, Shapiro GI, D’Andrea AD. Homologous recombination deficiency: exploiting the fundamental vulnerability of ovarian cancer. Cancer Discov 2015;5:1137-54.
177. Dibitetto D, Widmer CA, Rottenberg S. PARPi, BRCA, and gaps: controversies and future research. Trends Cancer 2024;10:857-69.
178. Lu Z, Mao W, Yang H, et al. SIK2 inhibition enhances PARP inhibitor activity synergistically in ovarian and triple-negative breast cancers. J Clin Invest 2022;132:e146471.
179. Krumm A, Barckhausen C, Kücük P, et al. Enhanced histone deacetylase activity in malignant melanoma provokes RAD51 and FANCD2-triggered drug resistance. Cancer Res 2016;76:3067-77.
180. Sun D, Yu M, Li Y, et al. Histone deacetylase 2 is involved in DNA damage-mediated cell death of human osteosarcoma cells through stimulation of the ATM/p53 pathway. FEBS Open Bio 2019;9:478-89.
181. Gupta VG, Hirst J, Petersen S, et al. Entinostat, a selective HDAC1/2 inhibitor, potentiates the effects of olaparib in homologous recombination proficient ovarian cancer. Gynecol Oncol 2021;162:163-72.
182. Sun L, Liu Y, Guo X, et al. Acetylation-dependent regulation of core spliceosome modulates hepatocellular carcinoma cassette exons and sensitivity to PARP inhibitors. Nat Commun 2024;15:5209.
183. Roos WP, Krumm A. The multifaceted influence of histone deacetylases on DNA damage signalling and DNA repair. Nucleic Acids Res 2016;44:10017-30.
184. Geng L, Cuneo KC, Fu A, Tu T, Atadja PW, Hallahan DE. Histone deacetylase (HDAC) inhibitor LBH589 increases duration of gamma-H2AX foci and confines HDAC4 to the cytoplasm in irradiated non-small cell lung cancer. Cancer Res 2006;66:11298-304.
185. Marampon F, Megiorni F, Camero S, et al. HDAC4 and HDAC6 sustain DNA double strand break repair and stem-like phenotype by promoting radioresistance in glioblastoma cells. Cancer Lett 2017;397:1-11.
186. Cuttini E, Goi C, Pellarin E, Vida R, Brancolini C. HDAC4 in cancer: a multitasking platform to drive not only epigenetic modifications. Front Mol Biosci 2023;10:1116660.
187. Chen F, Chen L, Qin Q, Sun X. Salt-inducible kinase 2: an oncogenic signal transmitter and potential target for cancer therapy. Front Oncol 2019;9:18.
188. Walkinshaw DR, Weist R, Kim GW, et al. The tumor suppressor kinase LKB1 activates the downstream kinases SIK2 and SIK3 to stimulate nuclear export of class IIa histone deacetylases. J Biol Chem 2013;288:9345-62.
189. Liang T, Wang F, Elhassan RM, et al. Targeting histone deacetylases for cancer therapy: trends and challenges. Acta Pharm Sin B 2023;13:2425-63.
190. Shi MQ, Xu Y, Fu X, et al. Advances in targeting histone deacetylase for treatment of solid tumors. J Hematol Oncol 2024;17:37.
191. Hontecillas-Prieto L, Flores-Campos R, Silver A, de Álava E, Hajji N, García-Domínguez DJ. Synergistic enhancement of cancer therapy using HDAC inhibitors: opportunity for clinical trials. Front Genet 2020;11:578011.
192. Moran B, Davern M, Reynolds JV, Donlon NE, Lysaght J. The impact of histone deacetylase inhibitors on immune cells and implications for cancer therapy. Cancer Lett 2023;559:216121.
193. Rutherford KA, McManus KJ. PROTACs: current and future potential as a precision medicine strategy to combat cancer. Mol Cancer Ther 2024;23:454-63.
194. Noonan EJ, Place RF, Pookot D, et al. miR-449a targets HDAC-1 and induces growth arrest in prostate cancer. Oncogene 2009;28:1714-24.
195. Shen Q, Yao Q, Sun J, et al. Downregulation of histone deacetylase 1 by microRNA-520h contributes to the chemotherapeutic effect of doxorubicin. FEBS Lett 2014;588:184-91.
196. Roccaro AM, Sacco A, Jia X, et al. microRNA-dependent modulation of histone acetylation in Waldenstrom macroglobulinemia. Blood 2010;116:1506-14.
197. Li Y, Gao L, Luo X, et al. Epigenetic silencing of microRNA-193a contributes to leukemogenesis in t(8;21) acute myeloid leukemia by activating the PTEN/PI3K signal pathway. Blood 2013;121:499-509.
198. Kim Y, Kim H, Park H, et al. miR-326-histone deacetylase-3 feedback loop regulates the invasion and tumorigenic and angiogenic response to anti-cancer drugs. J Biol Chem 2014;289:28019-39.
199. Topper MJ, Vaz M, Chiappinelli KB, et al. Epigenetic therapy ties MYC depletion to reversing immune evasion and treating lung cancer. Cell 2017;171:1284-300.e21.
200. Blagitko-Dorfs N, Schlosser P, Greve G, et al. Combination treatment of acute myeloid leukemia cells with DNMT and HDAC inhibitors: predominant synergistic gene downregulation associated with gene body demethylation. Leukemia 2019;33:945-56.
201. Scotto L, Kinahan C, Douglass E, et al. Targeting the T-cell lymphoma epigenome induces cell death, cancer testes antigens, immune-modulatory signaling pathways. Mol Cancer Ther 2021;20:1422-30.
202. Mazzu YZ, Yoshikawa Y, Nandakumar S, et al. Methylation-associated miR-193b silencing activates master drivers of aggressive prostate cancer. Mol Oncol 2019;13:1944-58.
203. Chang Y, Guo H, Li X, et al. Development of a first-in-class DNMT1/HDAC inhibitor with improved therapeutic potential and potentiated antitumor immunity. J Med Chem 2024;67:16480-504.
204. Liu B, Liu X, Han L, et al. BRD4-directed super-enhancer organization of transcription repression programs links to chemotherapeutic efficacy in breast cancer. Proc Natl Acad Sci U S A 2022;119:e2109133119.
205. Wen S, He Y, Wang L, et al. Aberrant activation of super enhancer and choline metabolism drive antiandrogen therapy resistance in prostate cancer. Oncogene 2020;39:6556-71.
206. Shang S, Yang J, Jazaeri AA, et al. Chemotherapy-induced distal enhancers drive transcriptional programs to maintain the chemoresistant state in ovarian cancer. Cancer Res 2019;79:4599-611.
207. Biehs B, Dijkgraaf GJP, Piskol R, et al. A cell identity switch allows residual BCC to survive Hedgehog pathway inhibition. Nature 2018;562:429-33.
208. Lai TH, Ozer HG, Gasparini P, et al. HDAC1 regulates the chromatin landscape to control transcriptional dependencies in chronic lymphocytic leukemia. Blood Adv 2023;7:2897-911.
209. Nguyen TTT, Zhang Y, Shang E, et al. HDAC inhibitors elicit metabolic reprogramming by targeting super-enhancers in glioblastoma models. J Clin Invest 2020;130:3699-716.
210. Gryder BE, Wu L, Woldemichael GM, et al. Chemical genomics reveals histone deacetylases are required for core regulatory transcription. Nat Commun 2019;10:3004.
Cite This Article
How to Cite
Download Citation
Export Citation File:
Type of Import
Tips on Downloading Citation
Citation Manager File Format
Type of Import
Direct Import: When the Direct Import option is selected (the default state), a dialogue box will give you the option to Save or Open the downloaded citation data. Choosing Open will either launch your citation manager or give you a choice of applications with which to use the metadata. The Save option saves the file locally for later use.
Indirect Import: When the Indirect Import option is selected, the metadata is displayed and may be copied and pasted as needed.
About This Article
Copyright
Data & Comments
Data
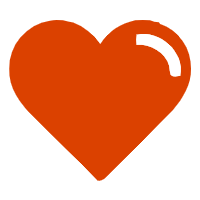
Comments
Comments must be written in English. Spam, offensive content, impersonation, and private information will not be permitted. If any comment is reported and identified as inappropriate content by OAE staff, the comment will be removed without notice. If you have any queries or need any help, please contact us at support@oaepublish.com.