Fra-1 affects chemotherapy sensitivity by inhibiting ferroptosis in gastric cancer cells
Abstract
Aim: Gastric cancer (GC) is one of the common malignant tumors, and most patients with advanced GC often develop chemotherapy resistance, resulting in poor chemotherapy efficacy. Therefore, it is crucial to clarify the specific mechanisms of their chemotherapy resistance.
Methods: In this study, we analyzed the correlation between fos-related antigen-1 (Fra-1) and chemotherapy resistance in GC using bioinformatics, cell counting kit-8 (CCK8), and 5-ethynyl-2’-deoxyuridine (EDU) combined with flow cytometry; furthermore, we used energy metabolomics sequencing, combined with ChIP-qPCR technology, to elucidate the specific role of Fra-1 in chemotherapy resistance of GC cells and its related mechanisms.
Results: We found that high Fra-1 expression was closely related to chemotherapeutic drugs in GC cells, as demonstrated by bioinformatics analysis combined with EDU and CCK8 experiments. Energy metabolomics combined with in vitro cellular experimental analysis revealed that the pentose phosphate pathway (PPP) was activated in GC cells with high Fra-1 expression, along with an increase in the synthesis of metabolites such as nicotinamide adenine dinucleotide phosphate (NADPH) and glutathione (GSH), a decrease in the level of reactive oxygen species (ROS), and the inhibition of their ferroptosis. In addition, ChIP-qPCR experiments confirmed that Fra-1 binds to the promoter of glucose-6-phosphate dehydrogenase (G6PD), a key rate-limiting enzyme of the PPP, and transcriptionally regulates its expression, which in turn activates the PPP and promotes chemotherapy resistance in GC cells.
Conclusion: Our research findings suggest that Fra-1 activates the PPP by upregulating G6PD transcriptional activity and inhibiting its ubiquitination level, inhibiting ferroptosis in GC cells and inducing chemoresistance. This provides an experimental basis for screening potential molecular targets for chemotherapy resistance in GC patients.
Keywords
INTRODUCTION
Gastric cancer (GC) ranks among the most prevalent malignant tumors globally, characterized by a low rate of early diagnosis and a dismal prognosis[1]. Chemotherapy stands as a primary treatment modality for the majority of patients with advanced GC. However, owing to the development of drug resistance, chemotherapy often yields poor efficacy, leading to a low 5-year survival rate among GC patients[2]. Chemotherapy resistance stems from multifaceted factors, resulting in a lack of specificity in target recognition[3]. Among these factors, ferroptosis emerges as a pivotal player in chemotherapy resistance. Ferroptosis represents a programmed cell death triggered by the accumulation of lipid peroxides and subsequent membrane damage. Activation of ferroptosis-related markers in tumor cells induces ferroptosis, mitigating tumor chemotherapy resistance, thereby enhancing chemotherapy efficacy[4-6]. For instance, studies have identified that tumors exhibiting high TYRO3 gene expression manifest resistance to PD-1/PD-L1 blockade by impeding ferroptosis, resulting in resistance in syngeneic mouse models and patients receiving anti-PD-1/PD-L1 therapy[7,8]. These findings underscore the close association between ferroptosis and tumor chemotherapy resistance. Notably, there exist pertinent reports on ferroptosis and chemotherapy resistance in GC. For instance, cancer-associated fibroblasts impede ferroptosis in GC and foster chemotherapy resistance through the secretion of miR-522[9,10]. In summary, ferroptosis exerts a pivotal role in acquired drug resistance in GC. Thus, elucidating the role and mechanism of ferroptosis in GC chemotherapy resistance holds profound significance for enhancing the efficacy and prognosis of GC patients.
Fos-related antigen-1 (Fra-1), a member of the activator protein-1 (AP-1) transcription factor family, exhibits heightened expression in various tumors, correlating closely with cellular processes[11-14]. Our previous investigations have unveiled a robust expression of Fra-1, impacting the cell cycle distribution and apoptosis of GC cells, thereby contributing to the initiation and advancement of GC[15]. Furthermore, through immunoprecipitation coupled with liquid chromatography-tandem mass spectrometry, our group identified a novel interacting protein, YWHAH, associated with Fra-1. Subsequent mechanistic elucidation unveiled that YWHAH positively modulates Fra-1 expression, consequently activating the HMGA1/PI3K/AKT signaling cascade and fostering GC proliferation[16]. In essence, Fra-1 assumes a pivotal role in the malignant progression of GC. Nonetheless, to date, there exists a dearth of literature elucidating the involvement and underlying mechanisms of Fra-1 in GC chemotherapy resistance.
In this study, we have elucidated the underlying mechanism by which Fra-1 promotes chemotherapy resistance in GC. Our findings reveal that Fra-1 directly interacts with the promoter region of glucose-6-phosphate dehydrogenase (G6PD), the first rate-limiting enzyme in the pentose phosphate pathway (PPP), thereby transcriptionally upregulating its expression. Furthermore, Fra-1 enhances the stability of G6PD protein levels by inhibiting the ubiquitin-proteasome pathway. Consequently, this activation of the PPP leads to increased synthesis of the PPP metabolite nicotinamide adenine dinucleotide phosphate (NADPH) and a subsequent reduction in intracellular reactive oxygen species (ROS) levels. Ultimately, this cascade of events culminates in the inhibition of ferroptosis in GC cells, thereby conferring chemotherapy resistance in GC cells.
METHODS
Cell culture
The GC cell lines AGS and HGC27 were obtained from the Institute of Oncology, School of Basic Medical Sciences, Central South University, China. Both AGS and HGC27 cells were cultured in RPMI-1640 medium (Procell, PM150110, Wuhan, China) supplemented with 10% FBS (Procell, 164210-50, Wuhan, China). Chemosensitivity was evaluated in AGS and HGC27 cells treated with 10 μM cisplatin (CDDP) (MCE, 15663-27-1, New Jersey, USA), while ferroptosis was induced in AGS and HGC27 cells using 8 μM erastin (Selleck, S7242, Houston, USA). All cells were maintained at 37 °C with 5% CO2 and confirmed to be mycoplasma-negative.
Vector
The following vectors were used: PLVX-mCMV-ZsGreen-PGK-Puro-Fra-1, a lentiviral system expression vector; Fra1-pCDNA3.1-3xFlag-C, a eukaryotic expression vector; G6PD-pCDNA3.1-3xFlag-C, a eukaryotic expression vector; TRIM21-pCDNA3.1-3xFlag-C, a eukaryotic expression vector; PSPAX2, a lentivirus packaging vector; PMD2.G, a lentivirus packaging vector; G6PD wild-type and mutant luciferase reporter gene plasmids: pGL3-Basic-G6PD-promoter-WT, pGL3-Basic-G6PD-promoter-MT; and PRL-TK, a eukaryotic expression vector. All constructs were selected for ampicillin resistance and screened with puromycin. They were purchased from biotech companies and confirmed through DNA sequencing. Antibodies and reagents used in the study can be found in Tables 1 and 2.
Antibody information
Name | Catalog No. | Ordering company |
GAPDH | YM3029 | ImunnoWay |
IgG | sc-34665 | Santa Cruz Biotechnology |
Goat anti rat IgG | RS0002 | ImunnoWay |
Goat anti mouse IgG | RS0001 | ImunnoWay |
HA-tag | YM3003 | ImunnoWay |
Flag | YM3808 | ImunnoWay |
Fra-1 | YT1772 | ImunnoWay |
SLC7A11 | YT8130 | ImunnoWay |
GPX4 | YN3047 | ImunnoWay |
G6PD | YM0291 | ImunnoWay |
6PGD | YT3691 | ImunnoWay |
TKT | YT5938 | ImunnoWay |
TALDO | YT6489 | ImunnoWay |
Reagent information
Name | Catalog No. | Ordering company |
Cycloheximide | HY-12320 | MCE |
MG132 | S2619 | Selleck |
6AN | S9783 | Selleck |
Puromycin | IP1280 | Solarbio |
CDDP | 15663-27-1 | MCE |
Erastin | S7242 | Selleck |
Cell viability assay (CCK8)
Cell viability was assessed using the CCK-8 reagent (Yeasen, 40203ES76, Shanghai, China) following the manufacturer’s instructions. Cells were seeded into 96-well plates and treated as indicated. After treatment, the culture medium was replaced with 100 μL of fresh medium per well, followed by the addition of 10 μL of CCK-8 solution. After 2 h of incubation at 37 °C with 5% CO2, the absorbance was measured at 450 nm using a microplate reader.
Plasmid and siRNA transfection
For plasmid transfection, 2 μg of plasmid or shRNA was transfected into GC cells using polyplus transfection reagent (Sartorius Stedim Biotech, Aubagne, France) following the manufacturer’s instructions in six-well plates. Cells were harvested 48 h post-transfection for further analysis. For transient transfection of siRNA, 5 μL of siRNA was transfected into GC cells using polyplus transfection reagent according to the manufacturer’s instructions. A six-well plate was used as an example. The target sequence for siNC was 5’-ACGUGACACGUUCGGGAGAATT-3’, and for siFra-1 was 5’-GGAAGGAACTGACCGACTT-3’. All siRNAs were obtained from Guangzhou Ribobio Co., Ltd.
RT-qPCR
Total RNA was extracted from cells using TRIzol (NCM Biotech, Beijing, China) and reverse transcribed into complementary DNA (cDNA) using the RNA First Strand cDNA Synthesis Kit (Yugong Biotech, Jiangsu, China). RT-qPCR assays were performed using the RT-qPCR Kit (SYBR Green method) (Yugong Life, Jiangsu, China) according to the manufacturer’s instructions. Relative gene expression levels were calculated using the 2-ΔΔCt method with GAPDH as the internal reference[17]. Primer sequences are provided in Table 3.
Primer sequence
Gene name | Upstream and downstream primer sequences |
Fra-1 | Forward 5‘-CAGTGGATGGTACAGCCTCATTTC-3’ Reverse 5‘-GCAGTCTCCTGTTCACAAGGC-3’ |
G6PD | Forward 5‘-ACACCAGGTATTTTGATGAGGAG-3’ Reverse 5‘-TCAGGCCGTGCCGCTGGCCGAGTAG-3 |
6PGD | Forward 5‘-AGCTGGTTTGGATCTTCGGA-3’ Reverse 5‘-CAGGTCATCCCCAGAGTTGT-3’ |
TKT | Forward 5‘-AGTTCATGTCACGCTGGGTA-3’ Reverse 5‘-CAGCTTCAGGTCTCCTTGGA-3’ |
TALDO | Forward 5‘-GCAACCCTTCTTTGACAACATTTTT-3’ Reverse 5‘-ATTTCTTCTCTCAGACGCTCTCC-3’ |
SLC7A11 | Forward 5‘-GGCAGTGACCTTTTCTGAGC-3’ Reverse 5‘-TGTCAAAGGGTGCAAAACAA-3’ |
GPX4 | Forward 5‘-GTAACCAGTTCGGGAAGCAG-3’ Reverse 5‘-TGTCGATGAGGAACTGTGGA-3’ |
FTH1 | Forward 5‘-GGAGAGGGAACATGCTGAGA-3’ Reverse 5‘-TGTCGATGAGGAACTGTGGA-3’ |
G6PD1 | Forward 5‘-CTCACTTCTGGTTCTGACCCC-3’ Reverse 5‘-TGACAATATGCGTGGAGCGG-3’ |
G6PD2 | Forward 5‘-CCATGACCATGTTTGGTGTC-3’ Reverse 5‘-TGGACAGTAAGAGCGGAAGG-3’ |
G6PD3 | Forward 5‘-TGACTTCCAGCAACACTGCC-3 Reverse 5‘-GGCCTCTGACATCAGTCACAA-3’ |
G6PD4 | Forward 5‘-AATGGGGGTCATTTTTGTCA-3’ Reverse 5‘-TGCGAAGAAACTGGGAGAGA-3’ |
G6PD5 | Forward 5‘-TGGTCACCTCACTCCACTCA-3’ Reverse 5‘-TCTCCTGGGAGTTCCTGATG-3’ |
G6PD6 | Forward 5‘-CACCTGTGGTCCCAGCTACT-3’ Reverse 5‘-GACAGTTGCCTGATGGGTTC-3’ |
GAPDH | Forward 5‘-TCAAGAAGGTGGTGAAGCAGG-3’ Reverse 5‘-TCAAAGGTGGAGGAGTGGGT-3’ |
Western blot
Total proteins were extracted using RIPA lysis buffer (Beyotime) containing protease inhibitors, and protein concentrations were determined using a BCA protein assay kit (Thermo Fisher Scientific). Proteins were separated by SDS-PAGE and transferred to PVDF membranes (Millipore, Bedford, MA, USA). Membranes were blocked with 5% skimmed milk and then incubated with primary antibodies overnight at 4 °C. After incubation with secondary antibodies, protein bands were visualized using an ECL detection reagent (NCM).
ChIP-qPCR
ChIP assays were performed using the ChIP assay kit (Beyotime, P2078, Wuhan, China) following the manufacturer’s protocol. Chromatin immunoprecipitation was performed, and gene enrichment was detected using RT-qPCR.
Dual luciferase reporter assay
Luciferase activity was measured using a dual luciferase reporter assay (Uelandy, F6075S, Suzhou, China) according to the manufacturer’s instructions. Fluorescence values were determined using a microplate reader.
Co-IP
Co-IP assays were conducted by lysing cells and immunoprecipitating the target proteins using magnetic beads conjugated with specific antibodies. Protein interactions were detected by SDS-PAGE electrophoresis.
Immunohistochemistry
Immunohistochemistry was performed on FFPE sections from mouse subcutaneous tumor tissues using specific primary antibodies. The staining intensity was scored, and images were analyzed.
Nude mouse experiment
Twenty 4-week-old female B/C nude mice were ordered from Hunan Slake Jingda Laboratory Animals, Ltd. and maintained in pathogen-free conditions. All animal experiments were conducted in accordance with the guidance of the Animal Ethics Committee of Central South University and approved by the Experimental Animal Welfare Ethics Committee of Central South University (approval no. CSU-2023-0337; Changsha, China). First, 20 mice were randomly divided into 4 groups. Subcutaneous tumorigenic experiments were then performed (injection of 5 × 106 HGC27 cells successfully overexpressed Fra-1). Tumor size was monitored every 3 days using the formula: (longest and shortest diameter2) × 0.5[18]. Mice were euthanized after the last measurement, and the tumors were collected for further studies.
Statistical analysis
All experiments were repeated at least three times, and data were expressed as mean ± standard deviation. Statistical analyses were performed using GraphPad Prism 9, with Student’s t-test or ANOVA used for comparisons between groups. P < 0.05 was considered significant (ns, not significant, *P < 0.05, **P < 0.01,***P < 0.001, ****P < 0.0001).
RESULTS
Fra-1 is highly expressed in GC tissue and is closely related to chemotherapy resistance and poor prognosis in GC cells
To delve deeper into the mechanistic insights underlying Fra-1’s impact on GC progression, we initiated an integrated analysis utilizing the GEPIA2 database, where we observed elevated expression levels of Fra-1 in GC tissues [Figure 1A]. Subsequent analysis through the DRESIS database further corroborated this observation, indicating a positive correlation between Fra-1 expression and the malignancy of GC patients
Figure 1. Fra-1 is closely associated with chemoresistance in GC. (A) Fra-1 expression in the STAD dataset analyzed using box plots from the GEPIA2 database. T, tumor samples (n = 408 cases); N, normal samples (n = 211 cases); (B) Kaplan-Meier Plotter survival curves showing the distribution of OS in GC patients with high/low expression of Fra-1 in the STAD cohort; (C) Wayne plots demonstrating differentially expressed genes common to GC drug resistance datasets GSE192631, GSE236987, and GSE186205; (D-G) Cell proliferation ability of GC cells after overexpression/silencing of Fra-1 and treatment with CDDP detected using the CCK8 Cell Proliferation Detection Kit; (H-K) Cell proliferation ability of GC cells after overexpression/silencing of Fra-1 and treatment with CDDP (10 μM) detected using the EDU cell proliferation detection kit. Scale bar = 50 μm; (L) Statistical graph of semi-quantitative results of EDU fluorescence values in GC cells. All experiments were performed with three technical replicates. *P < 0.05; **P < 0.01; ***P < 0.001. Fra-1: Fos-related antigen-1; GC: gastric cancer; OS: overall survival; CDDP: cisplatin; CCK8: cell counting kit-8; EDU: 5-ethynyl-2’-deoxyuridine.
Fra-1 induces chemoresistance in GC cells by activating the PPP metabolic pathway
To elucidate the intricate mechanism underlying Fra-1-mediated chemoresistance in GC cells, we initiated Fra-1 overexpression in AGS GC cells, followed by energy metabolomics analyses. These analyses unveiled a notable impact of Fra-1 on the energy metabolic pathway within GC cells. Further exploration through differential metabolite analyses revealed a significant upregulation of metabolites associated with the PPP in Fra-1-overexpressing GC cells [Figure 2A and B, Supplementary Figure 1E].
Figure 2. Fra-1 activates the PPP pathway in GC cells. (A) Heatmap showing the relative abundance of non-targeted metabolites in the NC group versus the Fra-1 overexpression group in GC cells (n = 6); (B) Expression levels of PPP metabolites; (C-F) RT-qPCR analysis of key molecules in the PPP metabolism pathway after overexpression/knockdown of Fra-1 in GC cells AGS and HGC27; (G and H) Protein levels of key molecules in the metabolic pathway of the PPP after overexpression/knockdown of Fra-1 in GC cells AGS and HGC27 detected by western blot assay; (I and J) G6PD enzyme activity measured after overexpression/knockdown of Fra-1 in GC cells AGS and HGC27 using the G6PD enzyme activity kit; (K and L) NADPH content measured after overexpression/knockdown of Fra-1 in GC cells AGS and HGC27 using the NADPH kit; (M and N) ROS content measured after overexpression/knockdown of Fra-1 in GC cells AGS and HGC27 using the ROS kit. All experiments were performed with three technical replicates. *P < 0.05; **P < 0.01; ***P < 0.001;
To corroborate the aforementioned metabolomics findings. Firstly, we employed RT-qPCR to analyze the mRNA levels of key molecules involved in PPP metabolic pathway. The results unveiled a significant upregulation of mRNA levels of the oxidative branch key enzymes G6PD, 6-phosphogluconolactonase (6PGL), and 6-phosphogluconate dehydrogenase (6PGD) in Fra-1-overexpressing GC cells, while no statistically significant differences were observed in the mRNA levels of the non-oxidative branch key enzymes transaldolase (TALDO) and transketolase (TKT). Conversely, the knockdown of Fra-1 led to a decrease in the mRNA levels of the aforementioned oxidative branch key enzymes in both AGS and HGC27 cells, with notable reductions observed in 6PGL and 6PGD mRNA levels [Figure 2C-F]. Moreover, western blot analysis demonstrated a significant increase in the protein levels of G6PD and 6PGD upon Fra-1 overexpression, whereas their protein levels were notably reduced upon Fra-1 knockdown [Figure 2G and H, Supplementary Figure 1F and G]. We further investigated whether Fra-1 influences G6PD enzymatic activity. We observed a significant elevation in G6PD enzyme activity upon Fra-1 overexpression, while
Meanwhile, we found that compared to the NC group, the Fra-1 overexpression group exhibited a slower decrease in cell proliferation and lower sensitivity to CDDP. Importantly, the proliferation of GC cells significantly decreased upon overexpression of Fra-1 combined with 6AN treatment compared to the Fra-1 overexpression group alone. Moreover, the proliferation of GC cells was further reduced following Fra-1 silencing combined with 6AN treatment compared to silencing Fra-1 alone(P < 0.01) [Figure 3A-D, Supplementary Figure 1J]. Furthermore, we corroborated these findings using the EDU cell proliferation assay. Consistently, the proportion of proliferating cells was higher in the Fra-1 overexpression group, indicating tolerance to CDDP compared to the NC group. Remarkably, the proportion of proliferating cells significantly decreased in the Fra-1 overexpression group concurrently treated with 6AN compared to Fra-1 overexpression alone. Furthermore, the proportion of proliferating cells was further reduced in the Fra-1 silencing group concurrently treated with 6AN (4 μM) compared to Fra-1 silencing alone(P < 0.05)
Figure 3. Fra-1 induces chemoresistance in GC cells by activating the PPP pathway. (A-D) Cell proliferation ability of GC cells after overexpression/silencing of Fra-1, treatment with PPP metabolism pathway inhibitor 6AN (4 μM), and treatment with different concentrations of CDDP detected using the CCK8 kit; (E-H) Cell proliferation ability of GC cells after overexpression/silencing of Fra-1 and treatment with 6AN (4 μM), and treatment with CDDP (10 μM) detected using the EDU cell proliferation assay kit; (I) Statistical graph of semi-quantitative results of EDU fluorescence values of GC cells. All experiments were performed with three technical replicates. *P < 0.05; **P < 0.01; ***P < 0.001; ****P < 0.0001. Fra-1: Fos-related antigen-1; GC: gastric cancer; PPP: pentose phosphate pathway; CDDP: cisplatin; CCK8: cell counting kit-8; EDU: 5-ethynyl-2’-deoxyuridine.
Fra-1 binds to the promoter of G6PD to regulate its transcription and thus activate the PPP metabolic pathway
Through RT-qPCR assays, we observed that the mRNA level of G6PD was upregulated in GC cells upon overexpression of Fra-1 and downregulated upon silencing of Fra-1 [Figure 4A-D], indicating that Fra-1 positively regulates G6PD mRNA expression. Consequently, we hypothesized that Fra-1 may activate PPP metabolism by directly modulating G6PD expression. To explore this hypothesis, we initially predicted potential binding sites of Fra-1 within the G6PD promoter using the JASPAR database and identified six putative binding sites [Figure 4E and F]. To validate the binding of Fra-1 to the G6PD promoter region, we synthesized primers targeting the identified binding sites and conducted ChIP-qPCR assays. Our findings revealed that Fra-1 binds to the 6th site of the G6PD promoter in GC cells AGS and HGC27(P < 0.0001)
Figure 4. Fra-1 binds to the G6PD promoter and transcriptionally regulates its expression. (A-D) Effects of overexpression/silencing of Fra-1 on G6PD mRNA levels in GC cells AGS and HGC27 detected by RT-qPCR assay; (E) Schematic diagram of G6PD promoter binding motif with Fra-1 DNA; (F) Prediction of possible binding sites of Fra-1 with the G6PD promoter using the JASPAR database; (G and H) Validation of the binding site of Fra-1 to G6PD using ChIP-qPCR experiments; (I and J) Detection of the binding of Fra-1 to the 6th binding site of G6PD using wild-type and mutant dual luciferase reporter gene vectors for the 6th binding site of G6PD in GC cells AGS and HGC27. All experiments were performed with three technical replicates. ns, no significant difference. *P < 0.05; **P < 0.01; ***P < 0.001; ****P < 0.0001. Fra-1: Fos-related antigen-1; G6PD: glucose-6-phosphate dehydrogenase; GC: gastric cancer.
Fra-1 inhibits G6PD ubiquitination, stabilizes its protein level and activates the PPP metabolic pathway
The impact of Fra-1 on G6PD extends beyond its transcriptional regulation; it also influences G6PD protein levels and stability through mechanisms independent of its binding to the G6PD promoter. To investigate this further, we overexpressed Fra-1 in GC cells AGS and HGC27 and treated them with the protein synthesis inhibitor cycloheximide (CHX). Subsequently, western blot assays revealed that in the absence of CHX treatment (NC group), the expression of G6PD protein began to decline after 1 h, progressively decreasing with prolonged CHX exposure, indicating intracellular degradation of G6PD protein. Conversely, following Fra-1 overexpression, the rate of G6PD degradation was notably attenuated. Specifically, degradation commenced after 3 h of CHX treatment, and the stability of G6PD protein was substantially higher compared to the NC group. These findings suggest that Fra-1 may enhance the stability of G6PD protein by inhibiting its degradation [Figure 5A and B, Supplementary Figure 1K and L].
Figure 5. Fra-1 stabilizes G6PD protein expression levels and activates the PPP metabolic pathway by inhibiting the G6PD ubiquitin-proteasome pathway. (A and B) Overexpression of Fra-1 in GC cells AGS and HGC27, followed by treatment with the protein synthesis inhibitor CHX for various durations, and detection of G6PD protein expression levels using western blot assay; (C and D) Treatment of GC cells AGS and HGC27 with CHX for various durations, with the addition of the proteasome pathway inhibitor MG132 in the experimental group, followed by detection of G6PD protein expression levels using western blot assay; (E and F) Silencing of Fra-1 in GC cells AGS and HGC27, followed by treatment with MG132, and detection of G6PD protein expression levels using western blot assay; (G and H) Co-transfection of Fra-1 plasmid and ubiquitin plasmid with HA tag (HA-Ub) into GC cells AGS and HGC27, followed by treatment with MG132, and detection of G6PD ubiquitination using immunoprecipitation assay; (I and J) Detection of cell proliferation ability in GC cells AGS and HGC27 after silencing Fra-1, silencing Fra-1 while overexpressing G6PD, and treatment with CDDP for 24 h using the CCK8 Cell Proliferation Detection Kit; (K-N) Detection of cell proliferation ability in GC cells AGS and HGC27 after silencing Fra-1, silencing Fra-1 while overexpressing G6PD, and treatment with CDDP (10 μM) for 24 h using the EDU cell proliferation detection kit. All experiments were performed with three technical replicates. *P < 0.05; **P < 0.01; ***P < 0.001. Fra-1: Fos-related antigen-1; G6PD: glucose-6-phosphate dehydrogenase; PPP: pentose phosphate pathway; GC: gastric cancer; CHX: cycloheximide; CDDP: cisplatin; CCK8: cell counting kit-8; cell counting kit-8; EDU: 5-ethynyl-2’-deoxyuridine.
To further investigate whether G6PD protein degradation relies on the ubiquitin-proteasome pathway, we treated GC cells AGS and HGC27 with CHX while concurrently adding the MG132 for 4 h. Our results demonstrated that in the presence of MG132, the stability of G6PD proteins was notably enhanced compared to the NC group, with a significant reduction in the rate of protein degradation [Figure 5C and D, Supplementary Figure 1M and N]. These findings suggest that G6PD degradation is dependent on the proteasome pathway. Additionally, we silenced Fra-1 in AGS and HGC27 cells and treated them with MG132. Remarkably, the knockdown of Fra-1 led to a reduction in G6PD protein expression, which was restored upon the addition of MG132 [Figure 5E and F, Supplementary Figure 1O and P], providing further evidence that Fra-1 stabilizes G6PD protein expression by modulating the proteasome pathway. These results solidify the notion that Fra-1 regulates G6PD protein stability through the proteasome pathway. Protein degradation via the proteasome pathway often involves ubiquitin protein binding. To ascertain whether G6PD interacts with ubiquitin proteins, we transfected Fra-1 plasmid and HA-Ub into AGS and HGC27 cells, followed by MG132 treatment after 48 h. Subsequently, immunoprecipitation (IP) experiments revealed that G6PD bound to ubiquitin proteins in the NC group following concurrent MG132 treatment. Notably, the proportion of G6PD binding to ubiquitin proteins was significantly diminished after Fra-1 overexpression coupled with MG132 treatment [Figure 5G and H]. These results strongly suggest that G6PD undergoes degradation via the proteasome-dependent pathway through ubiquitin binding. In summary, our findings indicate that Fra-1 inhibits the ubiquitination of G6PD, thereby stabilizing its protein level and highlighting the regulatory role of Fra-1 in modulating G6PD protein degradation through the ubiquitin-proteasome pathway.
To further elucidate the role of G6PD in mediating the effects of Fra-1 on chemoresistance in GC cells, we silenced Fra-1 and simultaneously overexpressed G6PD in AGS and HGC27 cells. Subsequently, the cells were treated with varying concentrations of CDDP, and their proliferative ability was assessed using a CCK8 kit. Our results demonstrated that the proliferation ability of cells in the siFra-1 group decreased more rapidly, indicating higher sensitivity to CDDP compared to the siNC group. Moreover, compared to the siNC group, the siFra-1 group exhibited a faster decline in proliferative capacity and increased sensitivity to CDDP. Conversely, after silencing Fra-1 and overexpressing G6PD, the proliferation ability of GC cells significantly increased compared to the siFra-1 group [Figure 5I and J]. These findings suggest that Fra-1 may activate the PPP metabolic pathway by stabilizing the protein level of G6PD, consequently reducing the cell proliferation ability and enhancing resistance to CDDP in GC cells. Furthermore, we validated these results using the EDU cell proliferation assay. In AGS and HGC27 cells, the proportion of proliferating cells in the Fra-1 silencing group exhibited lower sensitivity to CDDP compared to the siNC group. Notably, the proportion of proliferating cells in the group where Fra-1 was silenced while G6PD was overexpressed was significantly higher compared to the siFra-1 group (P < 0.01) [Figure 5K-N]. These findings further confirm that Fra-1 diminishes the sensitivity of GC cells to CDDP by activating the PPP metabolic pathway through the stabilization of G6PD protein levels.
Fra-1 inhibits ferroptosis in GC cells and induces chemotherapy resistance by activating the PPP metabolic pathway
After elucidating the specific mechanism by which Fra-1 activates the PPP pathway, we aimed to delve deeper into how Fra-1 activation of the PPP pathway induces chemotherapy resistance in GC cells. We, using a ROS detection kit combined with flow cytometry analysis, observed that the ROS levels in GC cells overexpressing Fra-1 decreased, while ROS levels recovered after treatment with 6AN (P < 0.001)
Figure 6. Fra-1 inhibits ferroptosis and induces chemoresistance in GC cells by activating the PPP metabolic pathway. (A) Detection of ROS levels in GC cells AGS after overexpression of Fra-1, and overexpression of Fra-1 along with treatment with 6AN (4 μM), using a ROS detection kit; (B-G) Detection of the effect of Fra-1 on GSH, MDA, Fe2+ levels in GC cells AGS and HGC27 using GSH, MDA, Fe2+ ELISA assay kit after overexpression/silencing of Fra-1; (H-K) RT-qPCR assay to detect mRNA expression levels of ferroptosis negatively related factors GPX4, SLCA7A11, and FTH1 in GC cells AGS and HGC27 after overexpression/silencing of Fra-1; (L) Detection of protein expression levels of ferroptosis negatively related factors GPX4 and SLCA7A11 in GC cells AGS and HGC27 after overexpression/silencing of Fra-1 using western blot assay; (M-R) Detection of the effect of Fra-1 on GSH, MDA, Fe2+ content in GC cells AGS and HGC27 after overexpression of Fra-1, and overexpression of Fra-1 along with treatment with 6AN (4 μM), using GSH, MDA, Fe2+ ELISA assay kit; (S-V) Detection of cell proliferation ability in GC cells AGS and HGC27 after overexpression/silencing of Fra-1, overexpression/silencing of Fra-1 along with the addition of ferroptosis inducer erastin (8 μM), and treatment with CDDP
To elucidate whether Fra-1 mediates chemotherapy resistance in GC cells by suppressing ferroptosis, we modulated Fra-1 expression levels and administered erastin, a ferroptosis inducer, in conjunction with CDDP treatment. Employing EDU cell proliferation assays alongside flow cytometry analysis, we observed a notable increase in the proliferation rate of GC cells overexpressing Fra-1 compared to the NC group upon exposure to CDDP, indicative of reduced sensitivity to CDDP. Conversely, cells overexpressing Fra-1 and treated with erastin exhibited a substantial decrease in proliferation and heightened sensitivity to CDDP compared to the Fra-1 overexpression group alone. Furthermore, silencing Fra-1 led to a significant reduction in cell proliferation relative to the NC group, accompanied by heightened sensitivity to CDDP. Interestingly, simultaneous treatment with erastin and Fra-1 silencing further diminished cell proliferation and enhanced sensitivity to CDDP compared to Fra-1 silencing alone(P < 0.05) [Figure 6S-V, Supplementary Figure 2W and X]. These findings collectively suggest that Fra-1 promotes chemotherapy resistance in GC cells by suppressing ferroptosis.
In vivo experiments further confirm that Fra-1 affects chemoresistance and tumor progression in GC cells through activation of the PPP pathway
To further validate the impact of Fra-1 on chemoresistance and tumor progression in GC cells, we conducted in vivo experiments. Female B/C nude mice were utilized as study subjects. Subcutaneous injection of 5 × 106 GC HGC27 cells stably overexpressing Fra-1 into the right axilla of each nude mouse initiated tumor formation. Following three weeks of treatment, the mice were euthanized, and tumor tissues were excised, photographed, and recorded. Our findings revealed that the Fra-1 overexpression group exhibited larger tumor volumes and accelerated growth rates compared to the null group. CDDP treatment significantly reduced tumor volume; however, the Fra-1 + CDDP group displayed larger tumor volumes, enhanced growth rates, and decreased sensitivity to CDDP compared to the null + CDDP group (P < 0.01)
Figure 7. In vivo experiments confirmed that Fra-1 promotes GC proliferation. (A and B) Twenty 4-week-old female B/C nude mice were randomly divided into four groups: null group, Fra-1 overexpression group, null + CDDP group, and Fra-1 overexpression + CDDP group, each containing five nude mice. GC cells HGC27 overexpressing Fra-1 were inoculated into the right axilla of each mouse. Tumor size was measured every 3 days using the formula: length × width2 × 0.5. When the tumor volume reached 10 mm3, CDDP solution
DISCUSSION
GC ranks sixth in incidence rate and third in mortality, making it a significant contributor to cancer-related deaths worldwide[19]. Most patients are diagnosed in advanced stages, where chemotherapy becomes a primary treatment modality[20]. With the detailed mechanisms of chemotherapy resistance in GC remaining incompletely understood, the prognosis for advanced GC patients remains poor, with low 5-year survival rates[14,21-23]. Thus, elucidating the underlying mechanisms of chemotherapy resistance in GC is imperative. Malignant tumor chemotherapy resistance often involves the activation of oncogenes. Fra-1, a member of the Fos family, serves as a crucial oncogene implicated in various biological processes, including cell growth, differentiation, and apoptosis[24,25]. In our previous study, we established that Fra-1 significantly influences the biological behavior of GC cells, including apoptosis, thereby implicating its involvement in GC development. Here, we observed high expression of Fra-1 in GC tissue, correlating closely with chemotherapy resistance and poor prognosis. In vitro cytology experiments further validated Fra-1’s impact on chemotherapy resistance in GC cells. Our findings underscore the pivotal role of elevated Fra-1 expression in mediating chemoresistance in GC cells. The involvement of Fra-1 in tumor malignancy has been extensively reported in various cancers. For instance, in breast cancer, Fra-1 acts as a regulator of epithelial-mesenchymal transition (EMT) and metastasis, while also enhancing chemical sensitivity[26-28]. Similarly, in colorectal cancer, Fra-1 overexpression promotes invasive phenotypes[29,30]. Moreover, through mechanisms like the miR-134-SDS22 feedback loop, Fra-1 promotes ERK/JNK signaling, reducing chemical sensitivity in ovarian cancer cells[31]. Our study aligns with these findings, highlighting the pivotal role of Fra-1 in chemotherapy resistance in GC cells.
To elucidate the intricate mechanism underlying Fra-1-mediated chemoresistance in GC cells, we initiated Fra-1 overexpression in GC cells, followed by energy metabolomics analyses. The results found that the metabolites related to the PPP were significantly upregulated in fra-1-overexpressing GC cells. PPP, a metabolic pathway that complements glycolysis, is abnormally activated in various malignant tumors. It serves as the primary source of NADPH and plays a crucial role in meeting the synthetic metabolic demands of cancer cells while combating oxidative stress by clearing intracellular ROS through redox reactions[32-34]. Several PPP enzymes have emerged as potential targets for cancer treatment[35-37]. Research has highlighted the involvement of PPP metabolism in cancer cell chemotherapy resistance processes[38-40]. For instance, in breast cancer, Rac1 activation of aldolase A and ERK signaling triggers the non-oxidative PPP, enhancing nucleotide metabolism and shielding breast cancer cells from chemotherapy-induced DNA damage, thereby inducing chemotherapy resistance[9]. G6PD, the first key rate-limiting enzyme in PPP metabolism, is often dysregulated, leading to aberrant activation or silencing of PPP metabolic pathways[33]. Studies have shown that PIKE-A activation of Akt binds to STAT3, stimulating FYN and inducing STAT3 phosphorylation, thereby enhancing G6PD transcription, activating PPP metabolism, promoting DNA and NADPH synthesis, and inhibiting ROS production, ultimately fostering tumor growth[41]. Moreover, HPD, closely linked to G6PD transcription, enhances tyrosine breakdown metabolism, elevating acetyl CoA levels for histone acetylation, and promoting HDAC10 translocation to the cytoplasm through LKB1/AMPK signaling, thereby controlling histone acetylation and boosting G6PD transcription, PPP flux, and tumor progression[42]. To further elucidate whether Fra-1 induces chemoresistance in GC cells by activating the PPP metabolic pathway, we conducted a series of experiments in AGS and HGC27 GC cells. Our study demonstrates that high Fra-1 expression activates the PPP metabolic pathway in GC cells, boosting NADPH synthesis and reducing intracellular ROS levels. Additionally, Fra-1 directly binds to the G6PD promoter, transcriptionally activating the PPP metabolic pathway, aligning with previous research. Our findings underscore the role of Fra-1 in inducing chemotherapy resistance in GC cells by activating the PPP metabolic pathway. While our study highlights the significance of metabolic reprogramming in GC cell survival, further investigations are warranted to elucidate the potential involvement of other metabolic pathways in GC chemotherapy resistance.
After elucidating the specific mechanism by which Fra-1 activates the PPP pathway, we aimed to delve deeper into how Fra-1 activation of the PPP pathway induces chemotherapy resistance in GC cells. Our results show that the Fra-1 overexpression inhibited ferroptosis in GC cells. Ferroptosis, a regulated cell death pathway, has been implicated in tumor chemotherapy resistance, with studies revealing a negative correlation between PPP metabolism activation and ferroptosis. Activation of the PPP metabolic pathway leads to the synthesis of reduced NADPH, promoting GSH synthesis and conversion of coenzyme Q10 to coenzyme Q10H2, thereby inhibiting peroxide accumulation. This activation also triggers the GPX4/GSH and FSP1/CoQ10H2 pathways, further suppressing ferroptosis[43,44]. The interplay between PPP metabolism and ferroptosis in tumor development is significant, yet research on PPP metabolism’s regulation of ferroptosis remains limited. The results of this study suggest that Fra-1 reduces intracellular ROS levels by activating the PPP metabolic pathway, thereby shielding cells from oxidative stress damage. Prior research has established PPP as an effective pathway for clearing ROS, and ferroptosis is closely linked to intracellular oxidation levels. Hence, we hypothesized whether Fra-1-induced activation of the PPP pathway inhibits ferroptosis, consequently inducing chemotherapy resistance in GC cells. Our study reveals that Fra-1 indeed inhibits ferroptosis in GC cells by activating the PPP metabolic pathway, consequently inducing chemotherapy resistance. This finding was corroborated by in vivo experiments in nude mice. However, further investigation is warranted to elucidate the detailed mechanism of ferroptosis-induced chemotherapy resistance in GC cells, including whether it involves classical ferroptosis-related pathways such as GPX4 and SLC7A11. In summary, our findings demonstrate that Fra-1 binds to the G6PD promoter, transcriptionally regulating its expression, and inhibits G6PD ubiquitination degradation by suppressing the ubiquitin-proteasome pathway, thereby stabilizing G6PD protein levels. This activation of the PPP metabolic pathway inhibits ferroptosis in GC cells, ultimately inducing chemotherapy resistance. Moreover, we found a short OS in GC patients with high Fra-1 expression by Kaplan-Meier survival analysis, indicating that the expression level of Fra-1 has a close correlation with the prognosis of GC patients. Meanwhile, in order to further verify the correlation between high Fra-1 expression and GC progression through in vivo experiments, we performed subcutaneous tumor formation experiments in nude mice. The results showed that Fra-1-overexpressing GC cells had significantly increased volume and fast growth rate. This result further confirmed that Fra-1 overexpression is able to promote GC proliferation and may be a new potential prognostic marker in GC patients.
In this study, we used 6AN, an inhibitor of the PPP, to detect its effect on Fra-1-mediated chemoresistance in GC cells in vitro. The results confirmed that Fra-1-mediated chemoresistance in GC cells was significantly attenuated when the PPP was blocked with 6AN. However, while the in vitro experiments yielded promising results, they could not fully replicate the complex interactions in the tumor microenvironment in vivo. Therefore, further in vivo animal model validation and clinical trial evaluation are still needed to determine whether 6AN can be an effective target for chemoresistance in GC cells with high Fra-1 expression.
In summary, our study confirms that Fra-1 regulates its expression by binding to the G6PD promoter and inhibiting the ubiquitin-proteasome pathway, thus stabilizing the G6PD protein level. This action activates the PPP metabolic pathway, leading to increased synthesis of reduced equivalent NADPH, decreased intracellular ROS levels, and inhibition of ferroptosis in GC cells, ultimately inducing chemotherapy resistance. These findings position Fra-1 as a novel regulatory factor in chemotherapy resistance for GC, highlighting its role in mediating G6PD expression to promote tumor chemoresistance. Consequently, Fra-1 emerges as a promising potential target for the clinical treatment of GC.
DECLARATIONS
Authors’ contributions
Performed the experiments: Zeng F, Cao J, Chen Y, Tang J, He Q, Liao S, Liang L, Li W, Liu S
Analyzed the data: Zeng F, Cao J, Chen Y
Designed the study and wrote the manuscript: Zeng F, Luo G, Zhou Y
All authors read and approved the final manuscript.
Availability of data and materials
The rata data supporting the findings of this study are available within this article and its Supplementary Materials. Further data is available from the corresponding authors upon request.
Financial support and sponsorship
The present study was supported by the National Natural Sciences Foundation of China (82273219), the Hunan Provincial Natural Science Foundation (2024JJ6623), and the innovation project of Central South University [grant numbers 2024ZZTS0530] (for Zeng F).
Conflicts of interest
All authors declared that there are no conflicts of interest.
Ethical approval and consent to participate
All animal protocols were approved by the Experimental Animal Welfare Ethics Committee of Central South University (CSU-2023-0337).
Consent for publication
Not applicable.
Copyright
© The Author(s) 2024.
Supplementary Materials
REFERENCES
1. Guan WL, He Y, Xu RH. Gastric cancer treatment: recent progress and future perspectives. J Hematol Oncol 2023;16:57.
2. Davodabadi F, Sajjadi SF, Sarhadi M, et al. Cancer chemotherapy resistance: mechanisms and recent breakthrough in targeted drug delivery. Eur J Pharmacol 2023;958:176013.
3. Wong TL, Loh JJ, Lu S, et al. ADAR1-mediated RNA editing of SCD1 drives drug resistance and self-renewal in gastric cancer. Nat Commun 2023;14:2861.
4. Stockwell BR. Ferroptosis turns 10: emerging mechanisms, physiological functions, and therapeutic applications. Cell 2022;185:2401-21.
5. Wang Y, Wu X, Ren Z, et al. Overcoming cancer chemotherapy resistance by the induction of ferroptosis. Drug Resist Updat 2023;66:100916.
6. Jiang X, Stockwell BR, Conrad M. Ferroptosis: mechanisms, biology and role in disease. Nat Rev Mol Cell Biol 2021;22:266-82.
7. Jiang Z, Lim SO, Yan M, et al. TYRO3 induces anti-PD-1/PD-L1 therapy resistance by limiting innate immunity and tumoral ferroptosis. J Clin Invest 2021;131:e139434.
8. Qi R, Bai Y, Li K, et al. Cancer-associated fibroblasts suppress ferroptosis and induce gemcitabine resistance in pancreatic cancer cells by secreting exosome-derived ACSL4-targeting miRNAs. Drug Resist Updat 2023;68:100960.
9. Zhang H, Deng T, Liu R, et al. CAF secreted miR-522 suppresses ferroptosis and promotes acquired chemo-resistance in gastric cancer. Mol Cancer 2020;19:43.
10. Wang Y, Zheng L, Shang W, et al. Wnt/beta-catenin signaling confers ferroptosis resistance by targeting GPX4 in gastric cancer. Cell Death Differ 2022;29:2190-202.
11. He YY, Zhou HF, Chen L, et al. The Fra-1: novel role in regulating extensive immune cell states and affecting inflammatory diseases. Front Immunol 2022;13:954744.
12. Zeng F, He J, Jin X, et al. FRA-1: a key factor regulating signal transduction of tumor cells and a potential target molecule for tumor therapy. Biomed Pharmacother 2022;150:113037.
13. Gao S, Tan H, Gang J. Inhibition of hepatocellular carcinoma cell proliferation through regulation of the cell cycle, AGE-RAGE, and leptin signaling pathways by a compound formulation comprised of andrographolide, wogonin, and oroxylin A derived from Andrographis Paniculata(Burm.f.) Nees. J Ethnopharmacol 2024;329:118001.
14. Gao S, Tan H, Li D. Oridonin suppresses gastric cancer SGC-7901 cell proliferation by targeting the TNF-alpha/androgen receptor/TGF-beta signalling pathway axis. J Cell Mol Med 2023;27:2661-74.
15. He J, Zhu G, Gao L, et al. Fra-1 is upregulated in gastric cancer tissues and affects the PI3K/Akt and p53 signaling pathway in gastric cancer. Int J Oncol 2015;47:1725-34.
16. He J, Zeng F, Jin XI, et al. YWHAH activates the HMGA1/PI3K/AKT/mTOR signaling pathway by positively regulating Fra-1 to affect the proliferation of gastric cancer cells. Oncol Res 2023;31:615-30.
17. Harshitha R, Arunraj DR. Real-time quantitative PCR: a tool for absolute and relative quantification. Biochem Mol Biol Educ 2021;49:800-12.
18. Huang F, Pang J, Xu L, et al. Hedyotis diffusa injection induces ferroptosis via the Bax/Bcl2/VDAC2/3 axis in lung adenocarcinoma. Phytomedicine 2022;104:154319.
19. López MJ, Carbajal J, Alfaro AL, et al. Characteristics of gastric cancer around the world. Crit Rev Oncol Hematol 2023;181:103841.
20. Arai H, Nakajima TE. Recent developments of systemic chemotherapy for gastric cancer. Cancers 2020;12:1100.
21. Rattanasinchai C, Llewellyn BJ, Conrad SE, Gallo KA. MLK3 regulates FRA-1 and MMPs to drive invasion and transendothelial migration in triple-negative breast cancer cells. Oncogenesis 2017;6:e345.
22. Hoang VT, Matossian MD, La J, et al. Dual inhibition of MEK1/2 and MEK5 suppresses the EMT/migration axis in triple-negative breast cancer through FRA-1 regulation. J Cell Biochem 2021;122:835-50.
23. Luo YP, Zhou H, Krueger J, et al. The role of proto-oncogene Fra-1 in remodeling the tumor microenvironment in support of breast tumor cell invasion and progression. Oncogene 2010;29:662-73.
24. Henckels E, Prywes R. Fra-1 regulation of matrix metallopeptidase-1 (MMP-1) in metastatic variants of MDA-MB-231 breast cancer cells. F1000Res 2013;2:229.
25. Moquet-Torcy G, Tolza C, Piechaczyk M, Jariel-Encontre I. Transcriptional complexity and roles of Fra-1/AP-1 at the uPA/Plau locus in aggressive breast cancer. Nucleic Acids Res 2014;42:11011-24.
26. Casalino L, Talotta F, Matino I, Verde P. FRA-1 as a regulator of EMT and metastasis in breast cancer. Int J Mol Sci 2023;24:8307.
27. Lu D, Chen S, Tan X, et al. Fra-1 promotes breast cancer chemosensitivity by driving cancer stem cells from dormancy. Cancer Res 2012;72:3451-6.
28. Song D, He H, Sinha I, et al. Blocking Fra-1 sensitizes triple-negative breast cancer to PARP inhibitor. Cancer Lett 2021;506:23-34.
29. Liu H, Ren G, Wang T, et al. Aberrantly expressed Fra-1 by IL-6/STAT3 transactivation promotes colorectal cancer aggressiveness through epithelial-mesenchymal transition. Carcinogenesis 2015;36:459-68.
30. Yun SI, Hong HK, Yeo SY, Kim SH, Cho YB, Kim KK. Ubiquitin-specific protease 21 promotes colorectal cancer metastasis by acting as a Fra-1 deubiquitinase. Cancers 2020;12:207.
31. Wu J, Sun Y, Zhang PY, et al. The Fra-1-miR-134-SDS22 feedback loop amplifies ERK/JNK signaling and reduces chemosensitivity in ovarian cancer cells. Cell Death Dis 2016;7:e2384.
33. TeSlaa T, Ralser M, Fan J, Rabinowitz JD. The pentose phosphate pathway in health and disease. Nat Metab 2023;5:1275-89.
34. Lucke-Wold BP, Logsdon AF, Turner RC, Huber JD, Rosen CL. Endoplasmic reticulum stress modulation as a target for ameliorating effects of blast induced traumatic brain injury. J Neurotrauma 2017;34:S62-70.
35. Li M, He X, Guo W, et al. Aldolase B suppresses hepatocellular carcinogenesis by inhibiting G6PD and pentose phosphate pathways. Nat Cancer 2020;1:735-47.
36. Jia D, Liu C, Zhu Z, et al. Novel transketolase inhibitor oroxylin A suppresses the non-oxidative pentose phosphate pathway and hepatocellular carcinoma tumour growth in mice and patient-derived organoids. Clin Transl Med 2022;12:e1095.
37. Franceschi S, Lessi F, Morelli M, et al. Sedoheptulose kinase SHPK expression in glioblastoma: emerging role of the nonoxidative pentose phosphate pathway in tumor proliferation. Int J Mol Sci 2022;23:5978.
38. Paul S, Ghosh S, Kumar S. Tumor glycolysis, an essential sweet tooth of tumor cells. Semin Cancer Biol 2022;86:1216-30.
39. Dong S, Liang S, Cheng Z, et al. ROS/PI3K/Akt and Wnt/β-catenin signalings activate HIF-1α-induced metabolic reprogramming to impart 5-fluorouracil resistance in colorectal cancer. J Exp Clin Cancer Res 2022;41:15.
40. Sun M, Li L, Niu Y, et al. PRMT6 promotes tumorigenicity and cisplatin response of lung cancer through triggering 6PGD/ENO1 mediated cell metabolism. Acta Pharm Sin B 2023;13:157-73.
41. Sun M, Sheng H, Wu T, et al. PIKE-A promotes glioblastoma growth by driving PPP flux through increasing G6PD expression mediated by phosphorylation of STAT3. Biochem Pharmacol 2021;192:114736.
42. Shan C, Lu Z, Li Z, et al. 4-hydroxyphenylpyruvate dioxygenase promotes lung cancer growth via pentose phosphate pathway (PPP) flux mediated by LKB1-AMPK/HDAC10/G6PD axis. Cell Death Dis 2019;10:525.
43. Li E, Clarke J, Ashrafian H, Darzi A, Neves AL. The impact of electronic health record interoperability on safety and quality of care in high-income countries: systematic review. J Med Internet Res 2022;24:e38144.
Cite This Article

How to Cite
Download Citation
Export Citation File:
Type of Import
Tips on Downloading Citation
Citation Manager File Format
Type of Import
Direct Import: When the Direct Import option is selected (the default state), a dialogue box will give you the option to Save or Open the downloaded citation data. Choosing Open will either launch your citation manager or give you a choice of applications with which to use the metadata. The Save option saves the file locally for later use.
Indirect Import: When the Indirect Import option is selected, the metadata is displayed and may be copied and pasted as needed.
About This Article
Copyright
Data & Comments
Data
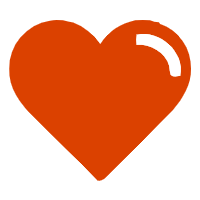
Comments
Comments must be written in English. Spam, offensive content, impersonation, and private information will not be permitted. If any comment is reported and identified as inappropriate content by OAE staff, the comment will be removed without notice. If you have any queries or need any help, please contact us at support@oaepublish.com.