Aging diastole - root cause for atrial fibrillation and heart failure with preserved ejection fraction
Abstract
The age-related decline in diastolic function can result in heart failure with a preserved ejection fraction (HFpEF) and atrial fibrillation (AF), which are comorbid conditions that are increasingly prevalent and have a high socioeconomic burden. In humans, diastolic dysfunction results from structural and functional changes that increasingly impede diastolic filling after midlife. Comorbidities and pathomechanisms that lead to additional increases in cardiac filling pressures accelerate the age-related deterioration in diastolic function. It is, therefore, that targeting the accelerators of diastolic dysfunction holds the most promise in reducing the risk for HFpEF and AF.
Keywords
INTRODUCTION
Diastolic dysfunction predisposes to heart failure with preserved ejection fraction (HFpEF) and atrial fibrillation (AF), which are prevalent, interdependent, and recurring comorbidities that reduce quality of life and survival. Both conditions share common risk factors, chief among them older age and the presence of hypertension, which adversely affect cardiac filling dynamics. We will first discuss the age-related changes in human cardiac structure and diastolic function and how they are modified. As the data contained in the literature often cover isolated findings or mechanisms, we rather focus on established patterns in aging that we deem most relevant to the deterioration in diastolic function. A better understanding of the intersecting pathophysiology of HFpEF and AF reveals therapeutic targets that can address both conditions.
BASIC CONSIDERATIONS
Diastole refers to the phase of the cardiac cycle when the heart relaxes and the chambers are refilled with blood, which is a key prerequisite to normal cardiac function. The atria are exposed to ventricular pressures for most of the cardiac cycle, which helps explain why AF and HFpEF are closely linked. Ventricular relaxation is not passive recoil but is governed by energy-consuming processes within cardiomyocytes that remove calcium ions from the myofilaments. This actively lengthens muscle fibers to create a lower-pressure environment that promotes ventricular filling by suction. Cellular regulatory mechanisms, such as the phosphorylation of proteins involved in muscle contraction, modify diastolic function greatly. In late diastole, atrial contraction boosts ventricular filling and results in a modest amount of passive ventricular distension. However, it is ultimately the arterial blood pressure that provides the necessary hydrostatic force to maintain positive venous and atrial pressures that allow ventricular filling.
The pressure in the left atrium is a surrogate for cardiac filling pressure that closely correlates with the cardinal symptom of impaired diastolic function, that is, shortness of breath. Changes in cardiac structure and the myocardial substrate, such as concentric hypertrophy and fibrosis, can adversely affect diastolic function. These relationships are further influenced by the varying circulatory demands, which change venous and arterial loads and are modulated by heart rate, the principal regulator of cardiac output. Cardiac output reserve is directly proportional to the ability to exercise, which is among the best predictors of life expectancy[1,2]. Before discussing the natural deterioration in diastolic function over a lifespan, we will review how diastolic function is assessed in clinical practice.
Assessment of diastolic function and HFpEF
The diagnosis of diastolic dysfunction and HFpEF is multifaceted and not straightforward. In 2022, the last AHA/ACC/HFSA practice guideline for the management of heart failure was released, replacing the 2013 guidelines[3,4]. In addition, in 2023, an expert consensus decision pathway for HFpEF was published[5]. These documents aimed to simplify the diagnosis and management of diastolic dysfunction and HFpEF.
What makes the diagnosis of HFpEF so difficult is that, despite its high prevalence, there is no perfect test to assess diastolic function. In patients with clinical symptoms consistent with volume overload or unexplained dyspnea, after a thorough history and physical exam, a transthoracic echocardiogram is the next step. If the ejection fraction is 50% or higher, HFpEF may be suspected. Important to the diagnosis of HFpEF is some evidence of increased filling pressures. Suggestive findings on echocardiography are the presence of enlarged atria, concentric ventricular remodeling, elevated pulmonary pressures, and a reduction in the tissue Doppler indices of relaxation[6]. However, evidence of diastolic dysfunction by echocardiography alone is insufficient to ascertain a diagnosis of HFpEF, and patients may still have HFpEF without abnormal diastolic function by echocardiography.
The gold standard for filling pressure elevations is a heart catheterization, which can be performed using one of two methods: right heart catheterization with pulmonary capillary wedge pressure measurement, or left heart catheterization with an assessment of left ventricular end-diastolic pressure. Increased filling pressures greater than 15 mmHg at rest, or if necessary with exercise (> 20 mmHg) or a fluid challenge
The most relevant cardiac biomarkers used for the clinical assessment of diastolic function and HFpEF are natriuretic peptides: B-type natriuretic peptide (BNP) and N-terminal pro-BNP (NT-proBNP). BNP and NT-proBNP (a by-product of the cleaved prohormone proBNP) are primarily released by the ventricles in response to increased wall tension and strain, first and foremost by pressure and/or volume overload[8]. The natriuretic peptides are hormones that modify salt and water homeostasis, as well as blood pressure, and have diuretic, natriuretic, and vasodilator properties. Heart failure is associated with increases in natriuretic peptide blood levels, and measuring them by a blood draw can help ascertain or exclude HFpEF. The degree of natriuretic peptide elevation is linked to the severity of heart failure and has prognostic implications[9]. Here, it is noteworthy that patients with HFpEF tend to have lower levels of natriuretic peptides than patients with heart failure and a reduced ejection fraction (HFrEF), especially if they are obese[8,10]. In other words, normal natriuretic peptide levels in an obese patient do not preclude diastolic dysfunction or HFpEF. On the contrary, levels may be elevated in senescence and renal dysfunction without clinical evidence of HFpEF.
Given that HFpEF is a clinical diagnosis, scoring systems have been developed to provide physicians with a diagnostic probability based on patient history, demographics, and objective testing. The two scoring systems are the H2FPEF and the HFA-PEFF algorithms[6,11]. The H2FPEF score includes six components scored between 1-3 points, and these include: heaviness (BMI > 30 kg/m2), hypertension (2 or more blood pressure medications), presence of AF or pulmonary hypertension (pulmonary artery systolic pressure
The HFA-PEFF algorithm, developed by the Heart Failure Association of the European Society of Cardiology, is more involved but includes guidance regarding patients with intermediate probabilities and additional advice on how to screen for diseases that mimic HFpEF[6]. The first step involves a patient’s pretest probability based on clinical signs and symptoms of heart failure, patient demographics, risk factors, and comorbidities, as well as findings on screening echocardiography. The second step involves extracting specific echocardiographic parameters and using natriuretic peptides to assign a score to each patient. These echocardiographic parameters include functional tests such as tissue Doppler velocities and the presence of pulmonary hypertension, as well as structural measures such as left atrial volume, left ventricular mass, and wall thickness. Natriuretic peptide levels are stratified based on the presence or absence of atrial fibrillation. Scores of 5 or greater are considered diagnostic for HFpEF. Scores of 2-4 have an intermediate probability for HFpEF and additional testing is recommended as a third step. These tests include a diastolic exercise stress echocardiogram or heart catheterization. Finally, step 4 is meant to exclude conditions that mimic HFpEF, such as infiltrative or restrictive cardiomyopathies. Additional testing may include advanced imaging, i.e., cardiac magnetic resonance imaging, cardiac computed tomography, positron emission tomography, or nuclear scintigraphy. Depending on the findings, endomyocardial biopsies may be warranted, and genetic testing may be advised. Once mimicking conditions are ruled out, the diagnosis of HFpEF can be made.
CONTRIBUTORS TO AGING-RELATED DIASTOLIC DYSFUNCTION
Changes in cardiac structure after midlife
It is commonly overlooked that normal aging is associated with an unfavorable structural remodeling of both atria and ventricles [Figure 1][12-15]. In midlife, left ventricular volumes start to regress by about 1% per year[13]. As ventricular mass does not change much with age, wall thickness increases, most notably in the basal septum, which reduces ventricular distensibility[12]. When combined with a lower chamber capacitance, this reduces functional reserve capacity and exercise tolerance[16]. In older adults, these changes are often detected by echocardiography, sometimes with evidence of diastolic dysfunction[17]. While ventricular volumes regress and diastolic function decreases with aging, atrial volumes increase primarily due to restricted ventricular filling[15]. These insidious and progressive changes not only reduce exercise capacity with aging but are also accompanied by measurable elevations in filling pressures that can manifest as dyspnea on exertion[18-21]. The increasing cardiac congestion contributes to venous remodeling with an associated expansion of the dependent venous blood pool that, when recruited to the central circulation during physical activity, overwhelms the heart rate-mediated contractile response with a build-up of diastolic myocardial tone that is pathognomonic for advanced diastolic dysfunction[22-28]. The resulting age-associated rise in cardiac filling pressure is the principal risk factor for AF and HFpEF. This process is hastened by arterial hypertension and reflected peripheral pressure waves that add to unfavorable structural remodeling[29]. This is evident in HFpEF patients, where smaller ventricular volumes are predictive of low exercise tolerance[30].
Exercise deficiency
Physical inactivity is an important contributor to age-related adverse cardiac remodeling, as it has been demonstrated that exercise is protective and can partially reverse ventricular volume loss and stiffness[16,31]. Additional evidence supporting the notion of exercise deficiency as an important contributor comes from echocardiographic studies of middle-aged patients after spinal cord injury. Compared to age-matched physically active individuals, calculated ventricular volumes are reduced by almost 20% among those with spinal cord injury, and the basal septum is thicker, which leads to reduced ventricular capacitance and compliance, notably in the absence of hypertension[32]. This increases the ventricular mass-to-volume ratio, which is a strong predictor of heart failure[13]. Although atrial dimensions were not reported in this study, early diastolic myocardial relaxation velocities were significantly reduced, suggestive of diastolic dysfunction, and explaining why paraplegic patients have a more than 3-fold increased risk for developing AF and heart failure[33,34]. A loss of left ventricular volumes has also been reported after prolonged space flight and extended bed rest, further supporting this notion[35].
In contrast, hypertensive heart disease manifests as ventricular hypertrophy with an increase in mass. The increased wall thickness can lead to additional reductions in ventricular volumes. When superimposed on age-related structural remodeling with a reduction in diastolic function, this can result in persistent and more pronounced elevations in filling pressures at rest and on exertion, reflective of heart failure. At this stage, some patients may start to feel the urge to elevate their chest position when recumbent, which reduces filling pressures, thereby alleviating shortness of breath. This explains why patients with advanced HFpEF frequently use several pillows at night or prefer sleeping in a reclining chair.
Electrophysiologic factors
The slowing in electrical conduction and subclinical sinus node dysfunction with aging also contribute to the decline in diastolic function and the progression of symptoms[36,37]. It is well established that the maximum predicted heart rate with exercise decreases markedly with age and the electrocardiographic
ACCELERATORS OF THE AGING-DEPENDENT IMPAIRMENT IN DIASTOLIC FUNCTION
Hypertension
As discussed, hypertensive heart disease is commonly associated with HFpEF and AF as it accelerates the progression of diastolic dysfunction from the codependent deterioration of the ventricular and atrial substrates superimposed on physiological aging [Figure 2][43]. This often manifests as an inappropriate rise in filling pressures on exertion or a volume challenge and is followed by sustained filling pressure elevations that permanently increase the atrial load[44].
Hypertension also directly contributes to increased filling pressures, and explains why HFpEF and AF in midlife are becoming more common and blood pressure reductions are beneficial[45,46]. The SPRINT hypertension trial, which compared a systolic blood pressure goal of 120 to 140 mmHg, was stopped prematurely for the benefits of the more intensive blood pressure reduction[47]. This benefit was predominantly driven by the decrease in incident heart failure [HR (95%CI) 0.62 (0.45-0.84)] with a separation of the cumulative primary event curves after one year. In other words, an optimal blood pressure of 120 mmHg prevents heart failure compared to a moderately elevated blood pressure of 140 mmHg. Unsurprisingly, lower blood pressures also resulted in less AF in the SPRINT trial [HR (95%CI) 0.74
Obesity-driven hypertension
The impact of obesity on blood pressure is strikingly obvious in the weight-loss trials of glucagon-like peptide 1 (GLP-1) agonists. For example, tirzepatide resulted in a 15% reduction in mean weight over
In clinical studies of HFpEF, about half of the patients have AF, and many with incident AF later develop HFpEF[55-58]. A majority of these patients have hypertension and receive blood pressure medications, and it is important to acknowledge that, despite the common use of antihypertensive agents, blood pressures in HFpEF outcome trials continue to be elevated[50,52,58].
More evidence for the propinquity of AF and obesity in contemporary HFpEF cohorts is embedded in the H2FPEF diagnostic score. The presence of AF weighs heaviest in predicting HFpEF, followed by a body mass index above 30 kg/m2[11]. It is unsurprising that the prevalence of hypertension (45%) and obesity (42%) are similar in the US. At present, overindulgence is the main accelerator of diastolic dysfunction and explains the rise in AF and HFpEF[58]. This is commonly described as the cardiometabolic HFpEF and AF phenotype.
Renal impairment-driven hypertension
In chronic renal disease, kidneys gradually lose their ability to effectively filter waste products and fluids. This results in fluid retention, causing an increase in blood volume and blood pressure that increases the risk for HFpEF and AF.
Chronic blood pressure elevations from any cause will eventually become detectable by cardiac imaging as hypertensive heart disease[43]. As discussed, the functional and structural ventricular and atrial abnormalities that reflect diastolic dysfunction include impaired atrial emptying, concentric left ventricular remodeling, or overt hypertrophy and left atrial dilation, to name a few[43,55]. Medication classes that are highly effective in HFrEF did not provide the same benefits in HFpEF, resulting in two decades of neutral clinical trials. This led to speculation that other pathomechanisms or conditions may have confounding roles in HFpEF[59]. The two most prominent non-blood pressure-driven conditions that may present as HFpEF will be discussed next.
Hypertrophic cardiomyopathy and cardiac amyloidosis
Hypertrophic cardiomyopathy (HCM) and cardiac amyloidosis (CA) also accelerate the age-dependent deterioration in diastolic function. Suspicion for either condition should be highest among younger HFpEF patients without a history of hypertension. It is also important to recognize that more than half of individuals after the age of 70 years will have microscopically detectable “senile” amyloid deposits of unknown clinical significance that, in all likelihood, will contribute to the age-dependent deterioration in diastolic function[29].
As stated earlier, when signs and symptoms of heart failure are present, due diligence is necessary to exclude both non-cardiac and cardiac mimickers of HFpEF. Of the cardiac causes, the most common mimickers include infiltrative or restrictive cardiomyopathies, pericardial diseases, or valvular heart disease. Echocardiography is a recommended starting point, whereas a “diagnostic shotgun” approach that employs every possible test is not helpful. A targeted approach is recommended that considers the clinical presentation within a patient's demographic and age range, and assessment of associated non-cardiac manifestations. After which, potential testing, which may include specific laboratory tests, advanced imaging, heart catheterization, and endomyocardial biopsy, can be considered[60].
HCM should be considered when there is unexplained left ventricular hypertrophy, especially with a family history of congestive heart failure or sudden cardiac arrest. Frequently, the diagnosis is suspected when left ventricular outflow tract obstruction or asymmetric hypertrophy is demonstrated by echocardiography. Additional cardiac magnetic resonance imaging can provide superior myocardial resolution with quantification of fibrosis, which adversely affects prognosis. Genetic testing can support the diagnosis in up to half the patients but also may have prognostic implications for affected individuals and their family members. Typically, endomyocardial biopsies have no role in the diagnosis of HCM.
CA is due to misfolded proteins that then form fibrils that infiltrate the myocardium. 95% of CA is either from misfolded mutant or wild-type transthyretin (TTR) protein, or light chain (AL) fragments from immunoglobulins and plasma cell dyscrasia[61]. While the incidence of AL CA is rather low (about 10 cases per million person-years), TTR CA can be rather common, possibly affecting about 13% of 60-year-olds with symptomatic HFpEF[62]. As amyloid is a systemic syndrome due to protein infiltration, extracardiac involvement is common and patients often present with a constellation of symptoms. Frequent signs and symptoms involve the musculoskeletal system, such as bicep tendon rupture, bilateral carpal tunnel syndrome, and lumbar spinal stenosis, to name a few. Patients with unexplained left ventricular hypertrophy, abnormal tissue Doppler on echocardiography, and low voltage electrocardiograms should be considered for CA screening, especially if extracardiac signs are present. Diagnosis of CA involves screening for AL disease with serum and urine protein immunofixation of antibody fragments, and performing bone scintigraphy, which can reveal an inappropriate myocardial uptake that is diagnostic for TTR disease. Treatment involves addressing the underlying cause, requiring a multidrug chemotherapy regimen for AL disease that may include autologous bone marrow transplant, or using protein stabilizers and silencers for wild-type or hereditary TTR disease.
In the next paragraph, we will critically reflect on mainstream research trends and misperceptions that, in our opinion, do not advance the understanding of diastolic dysfunction and are unlikely to result in treatments.
Etiologic distractors
HFpEF is a heterogeneous syndrome and it has been argued that non-cardiac factors and comorbidities are responsible for the many neutral trials[59,63]. We posit that the pathophysiology of HFrEF is even more heterogeneous and that the efficacy of sodium-glucose cotransporter-2 (SGLT-2) inhibitors across most HFpEF subpopulations substantially weakens the heterogeneity argument. It is true, however, that HFpEF, in large part due to age and hypertension as precipitating factors, has many comorbidities. Nevertheless, European and American funding agencies decided to prioritize research efforts into the phenotyping of HFpEF, which, in our opinion, will lead to a wealth of statistical associations but no actionable items that will actually benefit patients[64].
Efforts in the basic sciences to advance the understanding of HFpEF tend to focus on single, often arbitrary, mechanisms with the most innovative techniques, which for decades have not yielded a treatment for heart failure. At present, inflammation is commonly discussed as a cause for HFpEF, a hypothesized disorder of the myocardium and/or vasculature[65]. We are not aware of a clear link between inflammation and HFpEF. Additionally, in ventricular myocardium obtained from patients with HFpEF, there is no evidence that pro-inflammatory pathways and genes are activated, neither in the subendocardial myocardium nor in the epicardial myocardium[66,67]. The fact that obesity is generally accompanied by elevations in C-reactive protein should not be taken as evidence that inflammation causes diastolic dysfunction and HFpEF.
The track record of the publicly funded translational research enterprise is disappointing, to say the least, considering the large expense to taxpayers. Overbearing research bureaucracies, consensus-seeking review panels, and unproven selection mechanisms seem unable to turn discovery into health. To our knowledge, none of the proposed cures for rodent heart failure that utilized cutting-edge methods and were published in the most prestigious science journals were ever translated into a treatment. We surmise that artificial intelligence, the savior de-jour, may also not do the trick and we contend that normal human intelligence can prevail. A holistic view of the most promising treatment targets with multimodal therapeutic approaches that can address related defects will succeed or emerge by chance, as is the case with SGLT2 inhibitors.
In the following, we will discuss pathomechanistic themes and patterns that, based on available human data, play likely roles in the age-related deterioration of diastolic function to suggest realistic treatment targets.
DETERMINANTS OF DIASTOLIC DYSFUNCTION
Myocardial stiffness and fibrosis
As discussed, structural changes during normal aging alter the basic cardiac physical properties, which in itself will contribute to a rise in filling pressures. The changes in cardiac structure are paralleled by an age-related increase in myocardial fibrosis, predominantly by the increased deposition of collagen 1 and 3, that reduces tissue compliance to add to the progressive diminution in cardiac functional reserve[68,69]. Although the echocardiographic literature suggests an increase in ventricular mass with age, more accurate measurements by magnetic resonance imaging revealed the opposite, a small reduction in ventricular mass[13]. This discordance is explained by the age-related focal increase in basal septal thickness, which is a key measurement in mass estimation by echocardiography. Nevertheless, fibrosis increases with age, most likely in response to higher central blood pressures and, to a lesser extent, to replace lost cardiac myocytes. It is plausible that age-related arterial stiffening plays a contributing role by stimulating myocardial stretch mechanisms that initiate myokine signaling or activation of the angiotensin II/TGF-beta and endothelin-1 pathways that result in excess extracellular matrix production[68,70-72]. Other mechanisms that have been put forward are the effects of a more pro-inflammatory milieu in older hearts with radical oxygen species and the age-related decline in the turnover of collagen fibrils accompanied by increased cross-linking[40,73]. Such modified collagen may be less accessible to degradation mechanisms that balance extracellular matrix production and breakdown in response to demand-driven physiologic cardiac adaptations. It is clear from animal models and in patients that if hypertension is added to aging, fibrosis burden increases above what is normal for the respective age group[40]. In addition, hypertension will lead to a thickening of cardiac myocytes that at the organ level manifests as concentric hypertrophy with an increase in mass and stiffness. Left ventricular tissue samples of patients with hypertensive heart disease and overt HFpEF reveal a more than 3-fold increase in passive stiffness in stretched myocardium compared to age-matched control myocardium, with variable increases in total collagen expression and content[67,69,74].
Adrenergic signaling and protein hypophosphorylation
Another theme in aging and HFpEF is the attenuated adrenergic response leading to cellular hypophosphorylation[75]. Protein phosphorylation is a crucial biochemical process whereby kinases and phosphatases add or remove phosphate groups to cellular proteins. This process serves important functions such as regulation of enzyme activity, signal transduction, cellular communication, metabolic regulation, and gene expression. In the heart, basic functions such as contractility, sinus node activity, and conduction velocity are regulated by phosphorylation. Basal myocardial stiffness is also affected. A first clue that hypophosphorylation may play a role in HFpEF was provided in isolated myocytes of patients[76]. Administration of protein kinase A lowered passive stiffness more than in control cells. This effect was linked to titin, a giant cytoskeletal protein that changes its stiffness by phosphorylation[74,77]. Other indicators for the role of adrenergic hypophosphorylation in HFpEF come from the mild reduction in systolic function with prolonged contraction and relaxation indices, as well as the reduced responsiveness of the sinus node to adrenergic activation with either medications or exercise (chronotropic incompetence), which is improved by discontinuing beta-adrenergic antagonists[23,78-81]. Clinical studies tested the efficacy of phosphodiesterase type 3 (PDE3) inhibitors that slow the breakdown of cyclic AMP, with a subsequent increase in protein kinase A-dependent protein phosphorylation. These agents provided marked hemodynamic benefits in HFpEF, and improved the aforementioned clinical markers of hypophosphorylation, both at rest and during exercise[82-85]. It is, therefore, that PDE3 inhibitors can be viewed as adrenergic amplifiers that restore a more normal response that led to improvements in chronotropic response and 6-min walk distance, whereas amplification of the guanylyl cyclase pathway via PDE5 inhibition failed to exert a benefit[84,86]. The causes of the attenuated activation of the adrenergic pathway in humans are likely multifactorial and have not been specifically assessed and quantified. Phosphorylation also plays a key role in the regulation of cardiac contraction and relaxation, processes that are governed by myocyte calcium handling, which will be discussed next.
Prolonged relaxation
The pathognomonic prolongation of the velocity and time indices of cardiac relaxation in HFpEF, such as -dP/dT and tau, is primarily caused by a slowed speed of diastolic calcium removal from the myofilaments[87]. Changes in the speed of relaxation are largely accounted for by an altered cardiac sarcoplasmic reticulum (SR) calcium pump (SERCA2a) activity. Accelerated calcium sequestration into the SR shortens relaxation (lusitropic effect) while increasing the SR calcium content, which, upon release from the SR, will result in a stronger contraction (inotropic effect)[88]. SERCA2a activity is primarily modulated by the protein kinase A-mediated phosphorylation of the SR protein phospholamban (PLB)[89,90]. Therefore, the slowed relaxation kinetics in HFpEF are accompanied by a slowing of the contraction velocity and a reduction in systolic function that is clinically detectable[22,23,91]. A hastened SR calcium uptake by PLB phosphorylation, i.e., by the aforementioned PDE3 inhibitors, will accelerate relaxation and contraction. Faster removal of calcium from the myofilament enhances ventricular suction and will lower filling pressures in all chambers.
Calcium is also crucial in mediating the effects of heart rate on the myocardium. Higher rates accelerate contraction and relaxation dynamics, independent of adrenergic activation. This innate myocardial property, referred to as the Treppe effect or force‐frequency relationship, is the result of augmented cellular calcium cycling up to stimulation rates of 120-170 bpm, after which force development declines[92,93]. As the heart rate increases, so does relaxation velocity, which helps prevent incomplete relaxation. The myocardium of HFpEF patients abnormally retains cellular calcium, which adversely affects SR function, leading to prolonged relaxation and a build-up of diastolic tone[22,23]. While diastolic tone leads to smaller chamber volumes at rest, it is converted into higher filling pressures during exercise. Combined with chronotropic incompetence, these are key factors in the reduction of cardiac output reserve in HFpEF[24-26,94,95]. Aging generally leads to slower cellular calcium handling and relaxation through both SR-dependent and cell membrane mechanisms, as observed in humans and in experimental models. These changes are further compounded by the direct afterload-induced prolongation of relaxation in hypertension[71,96]. Varying cardiac cycle lengths, as the case in AF, will further perturb calcium handling. The inability to establish steady-state calcium handling may explain “cardiac irregulopathy”, and higher natriuretic peptide levels in AF[97]. As both contraction and relaxation are energy-consuming, it is plausible that the ability to exercise will be further limited by ischemia from coexistent coronary artery disease and microvascular rarefication[69]. Although prolonged relaxation and low heart rates are mechanistically linked, i.e., by hypophosphorylation, they have different pathomechanistic effects that independently contribute to diastolic dysfunction to warrant a separate discussion.
Below optimal heart rates and conduction system disease
As discussed earlier, latent and overt bradycardia leads to extended ventricular filling at higher pressures
There are several reasons why below-normal heart rates, i.e., due to age-related sinus node dysfunction, may play a role in the development of HFpEF and AF. First, the regressive age-related ventricular remodeling is not matched by higher heart rates, to maintain optimal filling dynamics[103]. Second, large outcome studies and analyses confirmed that pharmacological heart rate lowering increases the risk for HFpEF and AF[104-106]. Third, the impaired chronotropic response of the sinus node to adrenergic activation during exercise decreases with age[40,107]. It is revealing that hospitalized patients with HFpEF tend to present with substantially lower heart rates compared to HFrEF, even when corrected for the use of beta-blockers, suggesting a general propensity toward low heart rates that directly promote HFpEF and AF[95,108]. This is also reflected in the large HFpEF clinical trials, where the baseline heart rates are typically between 69-71 vs. 80 bpm in the general population[42,109]. In this context, it is rarely considered that heart rates are inversely related to body size, which explains why heart rate is higher in childhood and is about 5 bpm higher in women compared to men[110]. By ignoring this allometric relationship and accepting heart rates of 60 bpm as normal, individuals with smaller body habitus, i.e., women, can be chronically exposed to heart rate-mediated filling pressure elevations[42]. We have argued elsewhere that heart rates around 70 bpm, as observed in HFpEF trials, are likely too low for a majority of age-remodeled hearts that, based on their altered physical properties, would function more optimally at higher heart rates[111]. In other words, many older adults have concealed bradycardia despite not meeting current criteria[103]. It is, therefore, prudent to generally avoid beta-blockers in the treatment of patients with preserved ejection fractions whenever possible, as they will worsen filling dynamics and promote dyspnea, the cardinal symptom of HFpEF[39,99,112]. Only heart failure patients with sinus rhythm and a reduced ejection fraction derive an unequivocal and substantial benefit from selective beta-receptor blockade[113]. In the following section, we will discuss the treatment targets that appear most promising in overt diastolic dysfunction manifested as AF and HFpEF.
TREATMENT APPROACHES
AF treatment focuses on restoring sinus rhythm or controlling the ventricular rate. Because diastolic dysfunction is the prevailing cause for both HFpEF and AF, similar preventative strategies apply. However, most of the evidence basis for prevention has accrued in hypertension trials, such as the SPRINT trial[47].
Diuretic agents
Loop diuretics are a mainstay therapy to alleviate symptoms and prevent recurrent heart failure admissions. Although there is a lack of outcome studies, it is clear that dosing optimization in response to weight changes or signs and symptoms of heart failure improves outcomes. In a pivotal study of pulmonary artery pressure sensor-guided medication adjustments that predominantly centered around loop diuretics, 84 heart failure-related hospitalizations were reported in the pressure sensor intervention group (n = 270) compared with 120 in the control group (rate 0.32 vs. 0.44, hazard ratio [HR] 0.72, 95%CI: 0.60-0.85,
Antihypertensive agents
Given the prominent role of hypertension in the development of AF and HFpEF, blood pressure control is a central goal. Although there are no large comparative effectiveness trials, thiazides, angiotensin receptor blockers, angiotensin-converting enzyme inhibitors, or calcium channel blockers can be tailored to coexistent comorbidities. In our practice, we generally strive to normalize blood pressure. If this is not accomplished, we add mineralocorticoid receptor antagonists such as spironolactone to the regimen. The TOPCAT trial of spironolactone demonstrated a benefit in the participants from the Americas and finerenone confirmed this benefit[116,117]. If additional blood pressure control is required, we add sacubitril-valsartan to enhance blood pressure management and clinical efficacy, especially in patients with low normal ejection fractions[49]. If AF rate control is needed, i.e., heart rates that exceed 110 bpm, we avoid beta-blockers as they worsen diastolic function and preferentially use diltiazem or digoxin[112,118]. As discussed, in obesity-driven HFpEF and AF, it is already evident that GLP-1 agonists will be strikingly effective due to their significant ability to reduce blood pressure[54].
SGLT2 inhibitors
The first class of medications that demonstrated an unequivocal benefit in a majority of patients with HFpEF were the inhibitors of the renal sodium-glucose cotransporter 2 (SGLT2)[50,52]. These agents reduce the relative risk of HFpEF admissions by about 20% with minimal effect on mortality or surrogate markers of heart failure, such as quality of life. SGLT2 inhibitors have only minor blood pressure-reducing effects, and no single mechanism appears to fully explain their effectiveness.
Outlook over the next decade
Even if beta-blocker de-prescription could be more widely implemented and pharmacological advances that include SGLT-2 inhibitors and GLP-1 agonists for weight loss are fully adopted, the residual risk for HFpEF and AF will remain high as the incurred decrement in diastolic function and changes in cardiac structure are not fully reversible. This is already evident in patients who were obese earlier in life[119]. The changing demographics toward an older population will require the additional development of targeted treatments that counteract the progression of diastolic dysfunction and the underlying structural abnormalities.
PROSPECTIVE THERAPIES AND KNOWLEDGE GAPS
A rational treatment of diastolic dysfunction is provided by the aforementioned PDE3 inhibitors[120]. These agents amplify the muted adrenergic signaling by increasing the half-life of cAMP, which in turn activates protein kinase A, thereby restoring cellular phosphorylation. This not only hastens relaxation by accelerated myofilament calcium removal, but also reduces the stiffness of titin. In addition, heart rate is increased, which is closely associated with lower filling pressures, symptom burden, and improved exercise capacity[82,84,85]. There may be, however, some hesitance to engage in long-term studies, as the results of historical HFrEF trials are inappropriately extrapolated to HFpEF. In the group of patients with the most advanced HFrEF, long-term use of PDE3 inhibitors improved symptoms but was associated with a modest increase in mortality[121]. However, safety studies of related PDE3 inhibitors did not find higher death rates in other patient groups[122,123]. Conceptually related is personalized accelerated physiologic pacing, which augments calcium handling by increasing heart rates above normal[120,124]. This approach has a similar efficacy with the added benefit that it helps correct coexisting conduction system disease without relying on adrenergic activation [Figure 4][120,125]. Above-normal resting heart rates also induce a modest degree of stable eccentric remodeling with myocardial thinning[126]. This would improve not only ventricular capacitance but also compliance, which are prerequisites for a better exercise capacity[30,127]. This hemodynamic treatment approach also appears to unload the atria, which may reduce the propensity toward AF[125,128-130].
Figure 4. The combination of physiologic pacing, which recapitulates normal electrical conduction (short P wave and QRS duration) and enhances mechanical synchrony, and accelerated pacing, which abbreviates diastole and lowers filling pressures. The combination of physiologic and accelerated pacing has synergist effects.
It has been hypothesized and tested if an atrial shunt could provide a benefit by reducing left atrial pressures and symptoms. This approach, however, increases right-sided volume loads with unknown long-term effects and a risk of reproducing the untoward effects of naturally occurring atrial-level shunts. The clinical trials performed to date do not suggest a favorable effect in HFpEF and the benefits seen in subgroups may reverse with the aging-related progression of pulmonary resistance[131,132]. Similarly, splanchnic nerve denervation, which aims at reducing the excessive recruitment of dependent venous blood during exercise, has uncertain effects on cardiac performance and outcomes[133].
At present, several trials are testing the effectiveness of anti-inflammatory agents under the premise that inflammation is a significant driver of HFpEF, i.e., by activating pro-fibrotic pathways. Although anti-inflammatory therapy targeting the interleukin-1β innate immunity pathway was demonstrated to significantly lower the rate of recurrent vascular events, it did not benefit heart failure[134]. As discussed, inflammatory pathway activation is also not evident in myocardial biopsies of patients with HFpEF[66,67]. Therefore, suppressing inflammation is unlikely to improve diastolic function.
Due to the high prevalence of the obesity-driven cardiometabolic HFpEF phenotype, it is predictable that GLP-1 agonists will markedly reduce the risk for HFpEF and AF. These benefits are in large part conveyed by the blood pressure reduction, but it is conceivable that the para-adrenergic effect of GLP-1 agonists may provide an additional more targeted benefit, i.e., by increasing heart rates[135,136]. Within the conceptual framework of accelerated diastolic aging, we predict that diastolic function and congestion will not revert to a healthy baseline and only transiently improve with GLP-1 agonists. In other words, patients will continue to be at a higher risk for HFpEF and AF compared to age-matched individuals who were never obese.
Undoubtedly, many more factors contribute to the deterioration in diastolic function. We have highlighted the ones we deem clinically most relevant and supported by the accruing evidence. Except for hypertension, which is the #1 accelerator of diastolic dysfunction, it is not possible to rank them. We remain optimistic that better and more relevant research can add more nuance to our understanding of the natural deterioration of human diastolic function, which in turn should improve the odds of finding effective treatments. Caution is advised with proclamations of a cure, which, in our opinion, is as realistic as immortality.
SUMMARY
The age-dependent deterioration of diastolic function accelerated by obesity-driven hypertension is the main driver of the current HFpEF and AF epidemic. A better understanding of the shared pathophysiology will yield effective treatments that can ameliorate and possibly even slow the progression of diastolic dysfunction.
DECLARATIONS
Acknowledgments
The authors acknowledge the patients who have contributed to the understanding of how diastolic function can be modified to improve health and well-being.
Authors' contributions
Concept and manuscript draft, figures: Meyer M
Manuscript draft: Núñez J, Goyal P, Silverman DN
Concept and manuscript draft: van Berlo JH, Maharaj V
Availability of data and materials
Not applicable.
Financial support and sponsorship
Meyer M has received research funding from Medtronic and the Engdahl Family Foundation. van Berlo JH is supported by the National Heart, Lung, and Blood Institute (R01HL155993). Goyal P is supported by the National Institute on Aging grant K76AG064428.
Conflicts of interest
Meyer M and the University of Vermont have licensed patents for the use of pacemakers in the prevention and treatment of atrial fibrillation and heart failure with preserved ejection fraction. Núñez J has received honoraria for presentations or participation in advisory boards from Alleviant, AstraZeneca, Boehringer Ingelheim, Bayer, Novartis, Novo Nordisk, Pfizer, Roche, Rovi, and Vifor CSL (outside the submitted work). Goyal P has received consulting fees from Agepha Pharma, Akros Pharma, Axon Therapies, Bayer HealthCare Pharmaceuticals, and Sensorum Health, as well as personal fees for medicolegal consulting and expert testimony related to heart failure. And all other authors declared that there are no conflicts of interest.
Ethical approval and consent to participate
Not applicable.
Consent for publication
Not applicable.
Copyright
© The Author(s) 2024.
REFERENCES
2. Myers J, Prakash M, Froelicher V, Do D, Partington S, Atwood JE. Exercise capacity and mortality among men referred for exercise testing. N Engl J Med 2002;346:793-801.
3. Yancy CW, Jessup M, Bozkurt B, et al. 2013 ACCF/AHA guideline for the management of heart failure: a report of the American College of Cardiology Foundation/American Heart Association Task Force on practice guidelines. Circulation 2013;128:e240-327.
4. Heidenreich PA, Bozkurt B, Aguilar D, et al. 2022 AHA/ACC/HFSA guideline for the management of heart failure: executive summary: a report of the American College of Cardiology/American Heart Association Joint Committee on clinical practice guidelines. J Am Coll Cardiol 2022;79:1757-80.
5. Kittleson MM, Panjrath GS, Amancherla K, et al. 2023 ACC expert consensus decision pathway on management of heart failure with preserved ejection fraction: a report of the American college of cardiology solution set oversight committee. J Am Coll Cardiol 2023;81:1835-78.
6. Pieske B, Tschöpe C, de Boer RA, et al. How to diagnose heart failure with preserved ejection fraction: the HFA-PEFF diagnostic algorithm: a consensus recommendation from the Heart Failure Association (HFA) of the European Society of Cardiology (ESC). Eur Heart J 2019;40:3297-317.
7. Paulus WJ, Tschöpe C, Sanderson JE, et al. How to diagnose diastolic heart failure: a consensus statement on the diagnosis of heart failure with normal left ventricular ejection fraction by the heart failure and echocardiography associations of the European society of cardiology. Eur Heart J 2007;28:2539-50.
8. Iwanaga Y, Nishi I, Furuichi S, et al. B-type natriuretic peptide strongly reflects diastolic wall stress in patients with chronic heart failure: comparison between systolic and diastolic heart failure. J Am Coll Cardiol 2006;47:742-8.
9. Maisel AS, Krishnaswamy P, Nowak RM, et al. Rapid measurement of B-type natriuretic peptide in the emergency diagnosis of heart failure. N Engl J Med 2002;347:161-7.
10. Horwich TB, Hamilton MA, Fonarow GC. B-type natriuretic peptide levels in obese patients with advanced heart failure. J Am Coll Cardiol 2006;47:85-90.
11. Reddy YNV, Carter RE, Obokata M, Redfield MM, Borlaug BA. A simple, evidence-based approach to help guide diagnosis of heart failure with preserved ejection fraction. Circulation 2018;138:861-70.
12. Bluemke DA, Kronmal RA, Lima JA, et al. The relationship of left ventricular mass and geometry to incident cardiovascular events: the MESA (multi-ethnic study of atherosclerosis) study. J Am Coll Cardiol 2008;52:2148-55.
13. Cheng S, Fernandes VR, Bluemke DA, McClelland RL, Kronmal RA, Lima JA. Age-related left ventricular remodeling and associated risk for cardiovascular outcomes: the multi-ethnic study of atherosclerosis. Circ Cardiovasc Imaging 2009;2:191-8.
14. Lang RM, Badano LP, Mor-Avi V, et al. Recommendations for cardiac chamber quantification by echocardiography in adults: an update from the American society of echocardiography and the European association of cardiovascular imaging. J Am Soc Echocardiogr 2015;28:1-39.e14.
15. Zemrak F, Ambale-Venkatesh B, Captur G, et al. Left atrial structure in relationship to age, sex, ethnicity, and cardiovascular risk factors: MESA (multi-ethnic study of atherosclerosis). Circ Cardiovasc Imaging 2017;10:e005379.
16. La Gerche A, Howden EJ, Haykowsky MJ, Lewis GD, Levine BD, Kovacic JC. Heart failure with preserved ejection fraction as an exercise deficiency syndrome: JACC focus seminar 2/4. J Am Coll Cardiol 2022;80:1177-91.
17. Redfield MM, Borlaug BA. Heart failure with preserved ejection fraction: a review. JAMA 2023;329:827-38.
18. Borlaug BA, Nishimura RA, Sorajja P, Lam CS, Redfield MM. Exercise hemodynamics enhance diagnosis of early heart failure with preserved ejection fraction. Circ Heart Fail 2010;3:588-95.
19. Borlaug BA. Evaluation and management of heart failure with preserved ejection fraction. Nat Rev Cardiol 2020;17:559-73.
20. Wolsk E, Bakkestrøm R, Thomsen JH, et al. The influence of age on hemodynamic parameters during rest and exercise in healthy individuals. JACC Heart Fail 2017;5:337-46.
21. Zierath R, Claggett B, Arthur V, et al. Changes in pulmonary artery pressure late in life: the atherosclerosis risk in communities (ARIC) study. J Am Coll Cardiol 2023;82:2179-92.
22. Selby DE, Palmer BM, LeWinter MM, Meyer M. Tachycardia-induced diastolic dysfunction and resting tone in myocardium from patients with a normal ejection fraction. J Am Coll Cardiol 2011;58:147-54.
23. Runte KE, Bell SP, Selby DE, et al. Relaxation and the role of calcium in isolated contracting myocardium from patients with hypertensive heart disease and heart failure with preserved ejection fraction. Circ Heart Fail 2017;10:e004311.
24. Silverman DN, Rambod M, Lustgarten DL, Lobel R, LeWinter MM, Meyer M. Heart rate-induced myocardial Ca2+ retention and left ventricular volume loss in patients with heart failure with preserved ejection fraction. J Am Heart Assoc 2020;9:e017215.
26. Reddy YNV, Koepp KE, Carter R, et al. Rate-adaptive atrial pacing for heart failure with preserved ejection fraction: the RAPID-HF randomized clinical trial. JAMA 2023;329:801-9.
27. Molnár AÁ, Nádasy GL, Dörnyei G, et al. The aging venous system: from varicosities to vascular cognitive impairment. Geroscience 2021;43:2761-84.
28. Sorimachi H, Burkhoff D, Verbrugge FH, et al. Obesity, venous capacitance, and venous compliance in heart failure with preserved ejection fraction. Eur J Heart Fail 2021;23:1648-58.
29. Lakatta EG, Mitchell JH, Pomerance A, Rowe GG. Human aging: changes in structure and function. J Am Coll Cardiol 1987;10:42A-7A.
30. de la Espriella R, Palau P, Losito M, et al. Left ventricular volume and maximal functional capacity in heart failure with preserved ejection fraction: size matters. Eur J Heart Fail 2024.
31. Hieda M, Sarma S, Hearon CM Jr, et al. One-year committed exercise training reverses abnormal left ventricular myocardial stiffness in patients with stage B heart failure with preserved ejection fraction. Circulation 2021;144:934-46.
32. Driussi C, Ius A, Bizzarini E, et al. Structural and functional left ventricular impairment in subjects with chronic spinal cord injury and no overt cardiovascular disease. J Spinal Cord Med 2014;37:85-92.
33. Wang CC, Chang CT, Lin CL, Huang BR, Kao CH. Spinal cord injury is associated with an increased risk of atrial fibrillation: a population-based cohort study. Heart Rhythm 2016;13:416-23.
34. Yoo JE, Kim M, Kim B, et al. Increased risk of myocardial infarction, heart failure, and atrial fibrillation after spinal cord injury. J Am Coll Cardiol 2024;83:741-51.
35. Perhonen MA, Franco F, Lane LD, et al. Cardiac atrophy after bed rest and spaceflight. J Appl Physiol 2001;91:645-53.
36. Chow GV, Marine JE, Fleg JL. Epidemiology of arrhythmias and conduction disorders in older adults. Clin Geriatr Med 2012;28:539-53.
37. Mesquita T, Zhang R, Cho JH, et al. Mechanisms of sinoatrial node dysfunction in heart failure with preserved ejection fraction. Circulation 2022;145:45-60.
38. Andersen ØS, Krogh MR, Boe E, et al. Left bundle branch block increases left ventricular diastolic pressure during tachycardia due to incomplete relaxation. J Appl Physiol 2020;128:729-38.
39. Meyer M, LeWinter MM. Heart rate and heart failure with preserved ejection fraction: time to slow β-blocker use? Circ Heart Fail 2019;12:e006213.
41. Ostchega Y, Porter KS, Hughes J, Dillon CF, Nwankwo T. Resting pulse rate reference data for children, adolescents, and adults: United States, 1999-2008. Natl Health Stat Rep 2011;41:1-16.
42. Gillum RF. Epidemiology of resting pulse rate of persons ages 25-74-data from NHANES 1971-74. Public Health Rep 1992;107:193-201.
44. Borlaug BA, Melenovsky V, Russell SD, et al. Impaired chronotropic and vasodilator reserves limit exercise capacity in patients with heart failure and a preserved ejection fraction. Circulation 2006;114:2138-47.
45. Hall JE, do Carmo JM, da Silva AA, Wang Z, Hall ME. Obesity-induced hypertension: interaction of neurohumoral and renal mechanisms. Circ Res 2015;116:991-1006.
46. Russo C, Jin Z, Homma S, et al. Effect of obesity and overweight on left ventricular diastolic function: a community-based study in an elderly cohort. J Am Coll Cardiol 2011;57:1368-74.
47. Wright JT Jr, Williamson JD, Whelton PK, et al. A randomized trial of intensive versus standard blood-pressure control. N Engl J Med 2015;373:2103-16.
48. Soliman EZ, Rahman AF, Zhang ZM, et al. Effect of intensive blood pressure lowering on the risk of atrial fibrillation. Hypertension 2020;75:1491-6.
49. Solomon SD, McMurray JJV, Anand IS, et al. Angiotensin-neprilysin inhibition in heart failure with preserved ejection fraction. N Engl J Med 2019;381:1609-20.
50. Anker SD, Butler J, Filippatos G, et al. Empagliflozin in heart failure with a preserved ejection fraction. N Engl J Med 2021;385:1451-61.
51. Jastreboff AM, Aronne LJ, Ahmad NN, et al. Tirzepatide once weekly for the treatment of obesity. N Engl J Med 2022;387:205-16.
52. Solomon SD, McMurray JJV, Claggett B, et al. Dapagliflozin in heart failure with mildly reduced or preserved ejection fraction. N Engl J Med 2022;387:1089-98.
53. Jastreboff AM, Kaplan LM, Frías JP, et al. Triple-hormone-receptor agonist retatrutide for obesity - a phase 2 trial. N Engl J Med 2023;389:514-26.
54. Kosiborod MN, Abildstrøm SZ, Borlaug BA, et al. Semaglutide in patients with heart failure with preserved ejection fraction and obesity. N Engl J Med 2023;389:1069-84.
56. Meyer M, Du Fay Lavallaz J, Benson L, Savarese G, Dahlström U, Lund LH. Association between β-blockers and outcomes in heart failure with preserved ejection fraction: current insights from the SwedeHF registry. J Card Fail 2021;27:1165-74.
57. Goyal P, Kim M, Krishnan U, et al. Post-operative atrial fibrillation and risk of heart failure hospitalization. Eur Heart J 2022;43:2971-80.
58. Solomon SD, Vaduganathan M, Claggett BL, et al. Baseline characteristics of patients with HF with mildly reduced and preserved ejection fraction: DELIVER trial. JACC Heart Fail 2022;10:184-97.
59. Shah SJ, Kitzman DW, Borlaug BA, et al. Phenotype-specific treatment of heart failure with preserved ejection fraction: a multiorgan roadmap. Circulation 2016;134:73-90.
60. Kittleson MM, Ruberg FL, Ambardekar AV, et al. 2023 ACC expert consensus decision pathway on comprehensive multidisciplinary care for the patient with cardiac amyloidosis: a report of the american college of cardiology solution set oversight committee. J Am Coll Cardiol 2023;81:1076-126.
61. Sipe JD, Benson MD, Buxbaum JN, et al. Amyloid fibril proteins and amyloidosis: chemical identification and clinical classification International Society of Amyloidosis 2016 Nomenclature Guidelines. Amyloid 2016;23:209-13.
62. González-López E, Gallego-Delgado M, Guzzo-Merello G, et al. Wild-type transthyretin amyloidosis as a cause of heart failure with preserved ejection fraction. Eur Heart J 2015;36:2585-94.
63. Kitzman DW, Haykowsky MJ, Tomczak CR. Making the case for skeletal muscle myopathy and its contribution to exercise intolerance in heart failure with preserved ejection fraction. Circ Heart Fail 2017;10:e004281.
64. Shah SJ, Borlaug BA, Kitzman DW, et al. Research priorities for heart failure with preserved ejection fraction: national heart, lung, and blood institute working group summary. Circulation 2020;141:1001-26.
65. Paulus WJ, Tschöpe C. A novel paradigm for heart failure with preserved ejection fraction: comorbidities drive myocardial dysfunction and remodeling through coronary microvascular endothelial inflammation. J Am Coll Cardiol 2013;62:263-71.
66. Hahn VS, Knutsdottir H, Luo X, et al. Myocardial gene expression signatures in human heart failure with preserved ejection fraction. Circulation 2021;143:120-34.
67. Ye B, Bradshaw AD, Abrahante JE, et al. Left ventricular gene expression in heart failure with preserved ejection fraction-profibrotic and proinflammatory pathways and genes. Circ Heart Fail 2023;16:e010395.
68. Liu CY, Liu YC, Wu C, et al. Evaluation of age-related interstitial myocardial fibrosis with cardiac magnetic resonance contrast-enhanced T1 mapping: MESA (multi-ethnic study of atherosclerosis). J Am Coll Cardiol 2013;62:1280-7.
69. Mohammed SF, Hussain S, Mirzoyev SA, Edwards WD, Maleszewski JJ, Redfield MM. Coronary microvascular rarefaction and myocardial fibrosis in heart failure with preserved ejection fraction. Circulation 2015;131:550-9.
70. Fraticelli A, Josephson R, Danziger R, Lakatta E, Spurgeon H. Morphological and contractile characteristics of rat cardiac myocytes from maturation to senescence. Am J Physiol 1989;257:H259-65.
71. Janczewski AM, Lakatta EG. Modulation of sarcoplasmic reticulum Ca2+ cycling in systolic and diastolic heart failure associated with aging. Heart Fail Rev 2010;15:431-45.
73. Ferrucci L, Fabbri E. Inflammageing: chronic inflammation in ageing, cardiovascular disease, and frailty. Nat Rev Cardiol 2018;15:505-22.
74. Zile MR, Baicu CF, Ikonomidis JS, et al. Myocardial stiffness in patients with heart failure and a preserved ejection fraction: contributions of collagen and titin. Circulation 2015;131:1247-59.
75. Messias LR, Messias AC, de Miranda SM, et al. Abnormal adrenergic activation is the major determinant of reduced functional capacity in heart failure with preserved ejection fraction. Int J Cardiol 2016;203:900-2.
76. van Heerebeek L, Borbély A, Niessen HW, et al. Myocardial structure and function differ in systolic and diastolic heart failure. Circulation 2006;113:1966-73.
77. Borbély A, Falcao-Pires I, van Heerebeek L, et al. Hypophosphorylation of the stiff N2B titin isoform raises cardiomyocyte resting tension in failing human myocardium. Circ Res 2009;104:780-6.
78. Andersen MJ, Hwang SJ, Kane GC, et al. Enhanced pulmonary vasodilator reserve and abnormal right ventricular: pulmonary artery coupling in heart failure with preserved ejection fraction. Circ Heart Fail 2015;8:542-50.
79. Shah AM, Cikes M, Prasad N, et al. Echocardiographic features of patients with heart failure and preserved left ventricular ejection fraction. J Am Coll Cardiol 2019;74:2858-73.
80. Sarma S, Stoller D, Hendrix J, et al. Mechanisms of chronotropic incompetence in heart failure with preserved ejection fraction. Circ Heart Fail 2020;13:e006331.
81. Palau P, Seller J, Domínguez E, et al. Effect of β-blocker withdrawal on functional capacity in heart failure and preserved ejection fraction. J Am Coll Cardiol 2021;78:2042-56.
82. Nanayakkara S, Byrne M, Mak V, Carter K, Dean E, Kaye DM. Extended-release oral milrinone for the treatment of heart failure with preserved ejection fraction. J Am Heart Assoc 2020;9:e015026.
83. Kaye DM, Nanayakkara S, Vizi D, Byrne M, Mariani JA. Effects of milrinone on rest and exercise hemodynamics in heart failure with preserved ejection fraction. J Am Coll Cardiol 2016;67:2554-6.
84. Burkhoff D, Borlaug BA, Shah SJ, et al. Levosimendan improves hemodynamics and exercise tolerance in PH-HFpEF: results of the randomized placebo-controlled HELP trial. JACC Heart Fail 2021;9:360-70.
85. Aiad N, du Fay de Lavallaz J, Zhang MJ, et al. Cilostazol in patients with heart failure and preserved ejection fraction-The CLIP-HFpEF trial. ESC Heart Fail 2024.
86. Redfield MM, Chen HH, Borlaug BA, et al. Effect of phosphodiesterase-5 inhibition on exercise capacity and clinical status in heart failure with preserved ejection fraction: a randomized clinical trial. JAMA 2013;309:1268-77.
87. Zile MR, Baicu CF, Gaasch WH. Diastolic heart failure-abnormalities in active relaxation and passive stiffness of the left ventricle. N Engl J Med 2004;350:1953-9.
88. Eisner DA, Caldwell JL, Trafford AW, Hutchings DC. The control of diastolic calcium in the heart: basic mechanisms and functional implications. Circ Res 2020;126:395-412.
89. Luo W, Grupp IL, Harrer J, et al. Targeted ablation of the phospholamban gene is associated with markedly enhanced myocardial contractility and loss of beta-agonist stimulation. Circ Res 1994;75:401-9.
90. Meyer M, Bluhm WF, He H, et al. Phospholamban-to-SERCA2 ratio controls the force-frequency relationship. Am J Physiol 1999;276:H779-85.
91. Shah AM, Claggett B, Sweitzer NK, et al. Prognostic importance of impaired systolic function in heart failure with preserved ejection fraction and the impact of spironolactone. Circulation 2015;132:402-14.
92. Mulieri LA, Hasenfuss G, Leavitt B, Allen PD, Alpert NR. Altered myocardial force-frequency relation in human heart failure. Circulation 1992;85:1743-50.
93. Pieske B, Kretschmann B, Meyer M, et al. Alterations in intracellular calcium handling associated with the inverse force-frequency relation in human dilated cardiomyopathy. Circulation 1995;92:1169-78.
94. Tamargo M, Martínez-Legazpi P, Espinosa MÁ, et al. Increased chamber resting tone is a key determinant of left ventricular diastolic dysfunction. Circ Heart Fail 2023;16:e010673.
95. Kagami K, Obokata M, Harada T, et al. Diastolic filling time, chronotropic response, and exercise capacity in heart failure and preserved ejection fraction with sinus rhythm. J Am Heart Assoc 2022;11:e026009.
96. Leite-Moreira AF, Correia-Pinto J, Gillebert TC. Afterload induced changes in myocardial relaxation: a mechanism for diastolic dysfunction. Cardiovasc Res 1999;43:344-53.
97. Prinzen FW, Auricchio A, Mullens W, Linde C, Huizar JF. Electrical management of heart failure: from pathophysiology to treatment. Eur Heart J 2022;43:1917-27.
98. Williams Jr JF, Bristow MR, Fowler MB, et al. Guidelines for the evaluation and management of heart failure: report of the American College of Cardiology/American Heart Association Task Force on Practice guidelines (Committee on Evaluation and Management of Heart Failure). Circulation 1995;92:2764-84.
99. Meyer M, Rambod M, LeWinter M. Pharmacological heart rate lowering in patients with a preserved ejection fraction-review of a failing concept. Heart Fail Rev 2018;23:499-506.
100. Wilcox JE, Rosenberg J, Vallakati A, Gheorghiade M, Shah SJ. Usefulness of electrocardiographic QT interval to predict left ventricular diastolic dysfunction. Am J Cardiol 2011;108:1760-6.
101. Santos AB, Kraigher-Krainer E, Bello N, et al. Left ventricular dyssynchrony in patients with heart failure and preserved ejection fraction. Eur Heart J 2014;35:42-7.
102. Backhaus SJ, Lange T, Schulz A, et al. Cardiovascular magnetic resonance rest and exercise-stress left atrioventricular coupling index to detect diastolic dysfunction. Am J Physiol Heart Circ Physiol 2023;324:H686-95.
103. Infeld M, Wahlberg K, Cicero J, et al. Personalized pacing for diastolic dysfunction and heart failure with preserved ejection fraction: design and rationale for the myPACE randomized controlled trial. Heart Rhythm O2 2022;3:109-16.
104. Wachtell K, Lehto M, Gerdts E, et al. Angiotensin II receptor blockade reduces new-onset atrial fibrillation and subsequent stroke compared to atenolol: the Losartan intervention for end point reduction in hypertension (LIFE) study. J Am Coll Cardiol 2005;45:712-9.
105. Fox K, Ford I, Steg PG, Tardif JC, Tendera M, Ferrari R. Ivabradine in stable coronary artery disease without clinical heart failure. N Engl J Med 2014;371:1091-9.
106. Habel N, du Fay de Lavallaz J, Infeld M, et al. Lower heart rates and beta-blockers are associated with new-onset atrial fibrillation. Int J Cardiol Cardiovasc Risk Prev 2023;17:200182.
107. Xiao RP, Tomhave ED, Wang DJ, et al. Age-associated reductions in cardiac beta1- and beta2-adrenergic responses without changes in inhibitory G proteins or receptor kinases. J Clin Invest 1998;101:1273-82.
108. Lorenzo M, Miñana G, Palau P, et al. Lower heart rate in patients with acute heart failure: the role of left ventricular ejection fraction. Scand Cardiovasc J 2024;58:2386977.
109. Anker SD, Butler J, Filippatos G, et al. Baseline characteristics of patients with heart failure with preserved ejection fraction in the EMPEROR-preserved trial. Eur J Heart Fail 2020;22:2383-92.
110. Infeld M, Avram R, Wahlberg K, et al. An approach towards individualized lower rate settings for pacemakers. Heart Rhythm O2 2020;1:390-3.
111. Meyer M, Infeld M, Habel N, Lustgarten D. Personalized accelerated physiologic pacing. Eur Heart J Suppl 2023;25:G33-43.
112. Meyer M, Lustgarten D. Beta-blockers in atrial fibrillation-trying to make sense of unsettling results. Europace 2023;25:260-2.
113. Heidenreich PA, Bozkurt B, Aguilar D, et al. 2022 AHA/ACC/HFSA guideline for the management of heart failure: executive summary: a report of the American College of Cardiology/American Heart Association Joint Committee on clinical practice guidelines. JACC 2022;79:1757-80.
114. Abraham WT, Adamson PB, Bourge RC, et al. Wireless pulmonary artery haemodynamic monitoring in chronic heart failure: a randomised controlled trial. Lancet 2011;377:658-66.
115. The ALLHAT Officers and Coordinators for the ALLHAT Collaborative Research Group. Major outcomes in high-risk hypertensive patients randomized to angiotensin-converting enzyme inhibitor or calcium channel blocker vs diuretic: the antihypertensive and lipid-lowering treatment to prevent heart attack trial (ALLHAT). JAMA 2002;288:2981-97.
116. Pitt B, Pfeffer MA, Assmann SF, et al. Spironolactone for heart failure with preserved ejection fraction. N Engl J Med 2014;370:1383-92.
117. Solomon SD, McMurray JJV, Vaduganathan M, et al. Finerenone in heart failure with mildly reduced or preserved ejection fraction. N Engl J Med 2024;391:1475-85.
118. Kotecha D, Bunting KV, Gill SK, et al. Effect of digoxin vs bisoprolol for heart rate control in atrial fibrillation on patient-reported quality of life: the RATE-AF randomized clinical trial. JAMA 2020;324:2497-508.
119. Al Saikhan L, Chaturvedi N, Ghosh AK, Hardy R, Hughes A. Adulthood adiposity affects cardiac structure and function in later life. Eur Heart J 2024;45:3060-8.
120. Meyer M, LeWinter MM, Zile MR. A targeted treatment opportunity for HFpEF: taking advantage of diastolic tone. Circulation 2021;144:1269-71.
121. Packer M, Carver JR, Rodeheffer RJ, et al. Effect of oral milrinone on mortality in severe chronic heart failure. The PROMISE study research group. N Engl J Med 1991;325:1468-75.
122. Hiatt WR, Money SR, Brass EP. Long-term safety of cilostazol in patients with peripheral artery disease: the CASTLE study (Cilostazol: a study in long-term effects). J Vasc Surg 2008;47:330-6.
123. McHutchison C, Blair GW, Appleton JP, et al. Cilostazol for secondary prevention of stroke and cognitive decline: systematic review and meta-analysis. Stroke 2020;51:2374-85.
124. Infeld M, Cyr JA, Sánchez-Quintana D, et al. Physiological pacing for the prevention and treatment of heart failure: a state-of-the-art review. J Card Fail 2024;30:1614-28.
125. Infeld M, Wahlberg K, Cicero J, et al. Effect of personalized accelerated pacing on quality of life, physical activity, and atrial fibrillation in patients with preclinical and overt heart failure with preserved ejection fraction: the myPACE randomized clinical trial. JAMA Cardiol 2023;8:213-21.
126. Wahlberg KJ, Infeld M, Plante TB, et al. Effects of continuous accelerated pacing on cardiac structure and function in patients with heart failure with preserved ejection fraction: insights from the myPACE randomized clinical trial. J Am Heart Assoc 2024;13:e032873.
127. Klein FJ, Bell S, Runte KE, et al. Heart rate-induced modifications of concentric left ventricular hypertrophy: exploration of a novel therapeutic concept. Am J Physiol Heart Circ Physiol 2016;311:H1031-9.
128. Riaz S, McMahon SR, Stockey K, Meyer M, Weissler-Snir A. Effect of personalized accelerated pacing in patients with nonobstructive hypertrophic cardiomyopathy. J Am Coll Cardiol 2024;83:1492-3.
129. van Loon T, Rijks J, van Koll J, et al. Accelerated atrial pacing reduces left-heart filling pressure: a combined clinical-computational study. Eur Heart J 2024;45:4953-64.
130. Meyer M. Accelerated pacing as a treatment for heart failure with preserved ejection fraction and atrial fibrillation? Eur Heart J 2024;45:4965-7.
131. Shah SJ, Borlaug BA, Chung ES, et al. Atrial shunt device for heart failure with preserved and mildly reduced ejection fraction (REDUCE LAP-HF II): a randomised, multicentre, blinded, sham-controlled trial. Lancet 2022;399:1130-40.
132. Stone GW, Lindenfeld J, Rodés-Cabau J, et al. Interatrial shunt treatment for heart failure: the randomized RELIEVE-HF trial. Circulation 2024;150:1931-43.
133. Fudim M, Litwin SE, Borlaug BA, et al. Endovascular ablation of the right greater splanchnic nerve in heart failure with preserved ejection fraction: rationale, design and lead-in phase results of the REBALANCE-HF trial. J Card Fail 2024;30:877-89.
134. Ridker PM, Everett BM, Thuren T, et al. Antiinflammatory therapy with canakinumab for atherosclerotic disease. N Engl J Med 2017;377:1119-31.
135. Vila Petroff MG, Egan JM, Wang X, Sollott SJ. Glucagon-like peptide-1 increases cAMP but fails to augment contraction in adult rat cardiac myocytes. Circ Res 2001;89:445-52.
Cite This Article
How to Cite
Meyer, M.; Núñez J.; Goyal, P.; Silverman, D. N.; van Berlo, J. H.; Maharaj, V. Aging diastole - root cause for atrial fibrillation and heart failure with preserved ejection fraction. J. Cardiovasc. Aging. 2024, 4, 25. http://dx.doi.org/10.20517/jca.2024.22
Download Citation
Export Citation File:
Type of Import
Tips on Downloading Citation
Citation Manager File Format
Type of Import
Direct Import: When the Direct Import option is selected (the default state), a dialogue box will give you the option to Save or Open the downloaded citation data. Choosing Open will either launch your citation manager or give you a choice of applications with which to use the metadata. The Save option saves the file locally for later use.
Indirect Import: When the Indirect Import option is selected, the metadata is displayed and may be copied and pasted as needed.
About This Article
Copyright
Data & Comments
Data
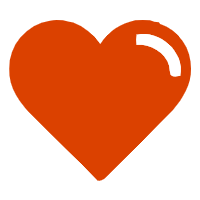
Comments
Comments must be written in English. Spam, offensive content, impersonation, and private information will not be permitted. If any comment is reported and identified as inappropriate content by OAE staff, the comment will be removed without notice. If you have any queries or need any help, please contact us at support@oaepublish.com.