The role of senescence in cancer therapy-associated cardiovascular toxicity
Abstract
Cardiovascular diseases (CVDs) and cancer are the two leading causes of global mortality. Cancer treatments, including radiotherapy and chemotherapy, can have severe cardiotoxic side effects, raising concerns for cancer patients and increasing the financial burden on healthcare systems. Recent studies have shown a link between cancer therapy-induced cardiotoxicity and cardiac senescence. Specifically, systemic cancer therapies are known to induce cardiac senescence, which may directly result in cardiac dysfunction or enhance the vulnerability of the heart to other stressors. Besides anthracyclines, newer, more targeted therapies such as tyrosine kinase inhibitors (TKIs) have also been shown to induce cardiac senescence. Cellular senescence is triggered by DNA damage, oncogene activation, reactive oxygen and nitrogen species, and other stressors, leading to the secretion of proinflammatory factors, increased oxidative stress, and disruption of normal cellular functions. Understanding the molecular mechanisms of cardiac senescence induced by cancer therapy is essential for attenuating or even preventing clinically overt cardiotoxicity using senotherapies such as senolytics and senomorphics. In this review, cancer therapies that are associated with CVDs are described with an emphasis on the potential role of cardiac senescence in the disease progression. In addition, the known mechanisms by which anthracyclines, particularly doxorubicin (DOX), radiotherapy, and TKIs lead to cardiac senescence are highlighted. Finally, recent and novel senotherapies for treating cellular senescence are discussed with a focus on targeting cardiac senescence following cancer treatment. The field remains in its early stages, with further research required to clarify how cancer treatments contribute to cardiotoxicity. At the same time, identifying senotherapies that can be safely combined with cancer drugs is essential for targeting cardiac senescence and protecting cardiac health in cancer patients.
Keywords
INTRODUCTION
Advances in cancer therapy, including the introduction of targeted treatments, have significantly improved cancer outcomes and increased the number of long-term survivors. However, these therapies can lead to serious cardiovascular side effects, which have become a major concern for cancer survivors[1]. In a United States population-based study involving 3.2 million cancer patients, 38% died from cancer while 11% died from cardiovascular diseases (CVDs), with the highest risk observed in the first year after diagnosis in patients under 85 years of age, particularly among patients under 35 years old[1]. Mostly affected were those with breast and prostate cancers, which are among the most frequent types diagnosed. Cardiotoxicity from cancer treatments can manifest years after treatment as hypertension, arrhythmias, coronary artery disease, and heart failure (HF), amongst others[1]. Commonly used agents, such as anthracyclines and tyrosine kinase inhibitors (TKIs), significantly increase the risk of HF, while radiation therapy is linked to various cardiovascular complications[2].
The mechanisms underlying cancer therapy-induced cardiotoxicity are complex and multifaceted. One notable mechanism is cellular senescence, a state of permanent cell cycle arrest typical of aging, which can be triggered by various cancer therapies[3]. Senescence can be initiated by DNA damage (from alkylating agents or radiation), activation of oncogenes (such as Ras and Myc), reactive oxygen and nitrogen species (ROS and RNS), and other stressors[4]. Senescent cells contribute to cardiotoxicity by secreting proinflammatory factors, promoting oxidative stress, and disrupting normal cellular functions[4]. In this review, we examine the features and mechanisms of senescence in cardiovascular health and disease, explore how cancer therapies induce cardiotoxicity through senescence, and discuss emerging anti-senescent therapies with the potential to treat cardiotoxicity induced by cancer treatment.
HALLMARKS OF CELLULAR SENESCENCE
Cellular senescence is a biological process that leads to a halt in proliferative capacity while maintaining the metabolic function of the cell in response to a variety of stressors[5]. It represents a double-edged sword process, playing a vital role in embryogenesis, development, tissue regeneration, and repair, as well as being a part of physiological aging. However, it is also a key mechanism in the development of many diseases[5]. Based on the inducer, senescence can be categorized into three main types: replicative, stress-induced premature senescence (SIPS), and oncogene-induced senescence (OIS)[6]. Replicative senescence is a hallmark of aging as it occurs due to telomere shortening and telomerase repression of somatic cells[7]. Telomeres, which are located at the ends of linear chromosomes, are crucial for genomic stability. In vertebrates, they consist of 5′-TTAGGG-3′ repeats bound by proteins that prevent the activation of a DNA damage response (DDR). However, since conventional DNA polymerases (such as Pol α and Pol δ) cannot fully replicate these ends without telomerase, telomeres shorten progressively with each cell division[8]. Once critically short, they lose sufficient capping proteins, exposing the DNA ends and activating DDR signaling pathways[9].
Inducers and mediators of senescent cells in the heart
The heart accumulates senescent cells during aging, as most of the cells that make up the heart are mitotic cells that are susceptible to replicative senescence[4]. In contrast, cardiomyocytes have very limited proliferative potential and are considered post-mitotic. Nevertheless, they can undergo a form of senescence called post-mitotic senescence[4]. During aging, the rate of senescent cell formation increases, while their clearance decreases, resulting in a significant accumulation of senescent cells[4]. Throughout aging, the heart experiences specific physiological and molecular alterations, including cardiomyocyte hypertrophy and fibrosis[4]. Studies have shown a correlation between accumulating cardiac senescent cells and CVDs such as atherosclerosis, ischemia, endothelial dysfunction, and vasomotor dysfunction[4]. Although replicative senescence induced by telomere shortening represents a physiological process in aging, it also occurs prematurely in patients suffering from CVDs due to several risk factors that induce SIPS. Sub-cytotoxic stressors, including cancer therapies like radiotherapy and chemotherapy, can trigger SIPS, leading to therapy-induced senescence, mitochondrial dysfunction, and immune dysregulation[6]. Those stressors expose the cell to chemical and physical stress, inducing irreversible growth arrest. Similar to replicative senescence, the accumulation of stress-induced senescent cells leads to CVDs, including HF, myocardial infarction (MI), hypertrophic cardiomyopathy, and fibrosis[10]. Additionally, OIS plays a significant role in CVDs by accelerating cellular aging and promoting inflammatory responses within the cardiovascular system. Aberrant activation of oncogenes and the downregulation of tumor suppressor genes such as p16 lead to a hyperproliferative state in cells, which results in proliferative exhaustion and growth arrest[11]. Although the induction of OIS ultimately suppresses cell proliferation and may work against malignant transformation, it can also promote cancer development. The net effect of OIS depends on the interplay of downstream signaling and the interaction of senescent cells with their microenvironment[12].
Characteristics of senescent cells
Even though the three main types of senescence (replicative senescence, SIPS and OIS) are triggered by different stimuli, they ultimately result in a common senescent phenotype. This phenotype is characterized by morphological alterations, macromolecular damage, metabolic changes, and a modified secretory profile, as depicted in Figure 1.
Figure 1. Mechanisms of cellular senescence. Senescence can be divided into different types including replicative senescence, oncogene-induced senescence, and stress-induced senescence. There are multiple different cellular inducers and mediators of senescence. The induction of senescence in a cell activates multiple different signaling mechanisms as a result of a DNA damage response (DDR), which inhibits cell cycle progression and results in a change in the morphology of the cell, as well as mitochondrial dysfunction, metabolic changes, and a senescence-associated secretory phenotype (SASP). In the pathways presented in the figure, the red "play" button indicates activation of the pathway. ETC: Electron transport chain; ROS: reactive oxygen species; ER: endoplasmic reticulum; SAHF: senescence-associated heterochromatin foci; CCF: cytoplasmic chromatin fragments. Created with BioRender.com software.
Morphological changes
A typical senescent cell shows an exceptionally flattened shape and enlarged morphology compared to a dividing cell. The enlarged and irregular morphology of the cell is not only confined to the cytosol but can also affect the nucleus. Senescent cells can also appear multinucleated, but more often, they exhibit an enlarged nuclear morphology, supported by the loss of lamin B1, utilized as a senescent-associated biomarker[13,14]. Lamins (lamin B1, B2, A, C) are components of the nuclear lamina, which consists of a fibrillar network underlying the inner nuclear membrane responsible for maintaining nuclear stability and chromatin organization[15,16]. It has also been reported that downregulation of lamin B1 occurring in senescent cells triggers senescence-associated heterochromatin foci (SAHFs) formation and dramatic chromatin reorganization[17,18]. The compromised integrity of the nuclear lamina in senescent cells upon lamin B1 downregulation contributes to the formation of cytoplasmic chromatin fragments (CCF), which are nuclear chromatin fragments that are shed into the cytoplasm[15,19] and enriched in epigenetic markers associated with an induction of the DDR through DNA damage[19,20].
Macromolecular damage
DNA damage such as DNA double-strand breaks, induces the recruitment of ataxia telangiectasia mutated (ATM) kinases to the DNA damage site[21], which triggers the phosphorylation of γ-H2A histone family member X (γ-H2AX) and thereby facilitates the recruitment of DNA damage repair factors including p53- binding protein-1(53BP1) and mediator of DNA damage checkpoint 1 (MDC1)[22]. 53BP1 and MDC1 are two important DDR components, which protect the stability of the genome. Therefore, their malfunction may contribute to the induction of tumorigenesis[23].
Senescence entry occurs via this ATM-induced phosphorylation of transcription factor p53, which activates p21CIP1 upon ATM/ataxia telangiectasia and rad3-related protein (ATR) stimulation, leading to cell cycle arrest or through p16 directly. p16INK4a maintains senescence by acting as a cyclin-dependent kinase (CDK) 4/6 inhibitor. It leads to retinoblastoma protein (RB) phosphorylation, causing the complex formation of RB-E2F, which induces cell cycle arrest through inhibited transcription of cell cycle genes[24,25]. Chromatin is not the only macromolecule affected by senescence. Indeed, other organelles, such as endoplasmic reticulum (ER) and mitochondria, involved in protein synthesis, stress sensing, and bioenergetics, are also affected. In senescent cells, different types of stress can cause ER dysfunctions, which induce an unfolded protein response (UPR). UPR is an adaptive response mechanism that reduces unfolded protein load to maintain cell viability and function. However, ER stress and UPR could also contribute to senescence through ER overactivity by the induction of oxidative stress. Oxidative stress and increased ROS production represent a hallmark of senescence, irrespective of the type of senescence: SIPS[26,27], replicative senescence[28], and OIS[28,29]. Similarly, mitochondrial dysfunction is a key feature of senescence and fuels multiple feedback loops that are responsible for inducing and maintaining the cellular senescence state. Mitochondrial dysfunction is defined as a decrease in the respiratory capacity per mitochondrion together with a decreased mitochondrial membrane potential, which typically can lead to electron transport chain (ETC) dysfunction and reduced nicotinamide adenine dinucleotide (NAD)+/NADH balance. Defects in the oxidative phosphorylation function have also been reported to occur in several models of senescence[30]. Aging and senescence cause mitochondrial dysfunction through several mechanisms, including mitochondrial DNA (mtDNA) mutations (genomic instability), mitochondrial turnover (defined as the ratio of mitochondrial biogenesis and mitophagy, associated with fusion and fission), nutrient signaling through mammalian target of rapamycin (mTOR), the NAD+/NADH ratio imbalance, and Ca2+ fluxes, resulting in mitochondrial Ca2+ overload[31].
Metabolic changes
Senescent cells also undergo significant metabolic reprogramming, which includes changes in mitochondrial function, lipid metabolism, and glucose metabolism. Key metabolic regulators, such as AMP-activated protein kinase (AMPK), sirtuins (SIRT1, SIRT2, SIRT3, SIRT6, SIRT7), FOXOs, and mammalian target of rapamycin (mTOR), play crucial roles in both driving and modulating senescence[32]. AMPK, which regulates glucose and fatty acid metabolism, is often inhibited in senescent myocardial tissues. However, its activation helps to maintain mitochondrial dynamics, alleviate ER stress and repress cardiomyocyte senescence[32]. Sirtuins, particularly SIRT2, regulate cardiomyocyte metabolism and reduce hypertrophy by activating the LKB1-AMPK signaling pathway[33]. In senescent cells, metabolic dysregulation is driven by factors such as NAD+ loss, excessive ROS production, mitochondrial dysfunction, and an imbalance in the NAD+/NADH ratio[34]. Mitochondrial dysfunction contributes to the senescence-associated phenotype, exacerbating pro-survival and anti-apoptotic pathways. These pro-survival mechanisms include the upregulation of BCL-2 family proteins and p53 pathways, which protect senescent cells from apoptosis[34]. Moreover, lipid metabolism in senescent cells is altered, with increased sphingomyelin-ceramide pathway activity and elevated levels of free polyunsaturated fatty acids. These are incorporated into triglycerides and accumulate as lipid droplets, typical of the senescent phenotype[35]. The breakdown of lipids and increased β-oxidation also play a role in maintaining cell cycle arrest[35]. Additionally, dysregulated autophagy and lysosomal function are hallmarks of senescence, with senescence-associated
Secretory phenotype
Senescent cells share a common secretory phenotype characterized by the release of several senescence-associated secretory phenotype (SASP) factors that enhance the secretory profile of senescent cells. These are mainly soluble factors (sSASP), including interleukins (IL) and cytokines, chemokines, growth factors, and proteases, which promote the clearance of senescent cells and wound healing after injury[38]. In addition, the release of bioactive lipids[39], free non-coding nucleic acids[40,41], and small extracellular vesicle SASP (eSASP)[40,42,43] factors has also been associated with senescent cells. However, the exact composition of SASP factors varies by cell type, tissue, and stimulus and this process is dynamic and temporally related. The most known components of the core secretome are: IL-1α, IL-1β, IL-6[44-53], chemokine IL-8, monocyte chemoattractant protein 1 (MCP1; also known as CCL2)[54-64], matrix metalloproteinases 1 and 3 (MMP1 and MPP3)[65], serine/cysteine proteinase inhibitors[66], and tissue inhibitors of metalloproteinases[38,67,68]. Recently, it has been discovered that bioactive lipids[39], small extracellular vesicles[40,42,43,69], and free non-coding nucleic acids[40,41] are also part of the SASP secretome[70]. Since SASP factors do not only act in an autocrine manner to enhance the senescent state, but also influence neighboring cells and organs through a paracrine action, they have both beneficial and detrimental effects[71,72]. In particular, the influence of senescent cells on the heart can have serious consequences for cardiovascular health, as will be discussed in the next sections.
SENESCENCE IN CARDIOVASCULAR HEALTH AND DISEASE
Cardiac tissue is composed of a variety of cell types, with cardiomyocytes and non-myocytes (fibroblasts, endothelial cells, and immune cells) playing distinct roles in cardiac function and pathogenesis[73]. Cardiomyocytes are highly specialized cells and post-mitotic, meaning they do not readily divide, while mitotic non-myocytes participate in tissue repair, extracellular matrix (ECM) maintenance, and immune responses[74]. Senescence in cardiac cell types is associated with the development of various CVDs, including MI and cardiac hypertrophy. In cardiomyocytes, senescence primarily impairs contractility and function in the heart, reducing its ability to compensate for its impaired function upon injury or stress. This dysfunction often results in maladaptive remodeling, contributing to hypertrophy - a compensatory increase in heart size[68]. In contrast, senescent non-myocytes such as fibroblasts can lead to excessive fibrosis, vascular stiffening, and chronic inflammation, thereby exacerbating heart failure after MI. The distinct contributions of these cell types underscore the complexity of senescence-driven CVDs[68]. Although MI and hypertrophy are both linked to senescence, they represent different pathological processes. MI typically involves acute ischemic damage, in which senescence halts tissue repair, leading to fibrosis and chronic inflammation. Hypertrophy, on the other hand, stems from long-term mechanical stress, driving compensatory growth that ultimately becomes maladaptive through senescence-induced tissue stiffening and dysfunction[68]. Understanding these processes and the role of senescence in the different cardiac cell types at molecular and cellular levels is critical to developing targeted therapies for senescence-related CVDs.
Cardiomyocytes
Cardiomyocytes are known to be mostly fully differentiated and post-mitotic in the adult mammalian heart. Consequently, cell cycle arrest is not a hallmark of senescence in this cell type[74]. However, recent evidence suggests that post-mitotic cells can also undergo senescence, contributing to tissue pathogenesis and disease[75]. Cardiomyocyte senescence can occur in response to aging, oxidative damage, DNA damage, and mitochondrial dysfunction - often triggered by distinct conditions such as MI or hypertrophic cardiomyopathy[76]. In MI, cardiomyocytes experience ischemia-reperfusion injury, resulting in significant mitochondrial damage and excessive production of ROS. This oxidative stress depletes cellular energy and exacerbates mitochondrial dysfunction, triggering senescence pathways that involve p53 and p16INK4a-Rb. The decline in mitochondrial function leads to a reduction in critical enzymes, such as AMPK, further contributing to maladaptive remodeling and cardiac dysfunction after MI[77,78]. In contrast, hypertrophy involves the enlargement of cardiomyocytes in response to increased workload or stress, such as hypertension. Hypertrophic growth of the heart, while initially adaptive, can lead to pathological remodeling and cardiac dysfunction over time. In patients with hypertrophic or dilated cardiomyopathy and in aged mice, cardiomyocytes have been shown to undergo telomere shortening, which induces senescence and promotes maladaptive changes[75]. Unlike other cell types, senescent cardiomyocytes from aged mice do not produce a classical SASP, but instead secrete factors such as endothelin 3, transforming growth factor β2 (TGF-β2), and growth differentiation factor 15, which can reduce fibroblast proliferation while activating myofibroblasts and promoting further cardiomyocyte hypertrophy[75]. The distinct mechanisms of cardiomyocyte senescence in MI vs. hypertrophy underscore different pathways of cardiac dysfunction, with mitochondrial damage and ROS signaling playing a larger role in MI, while telomere shortening drives hypertrophic changes.
Fibroblasts and myofibroblasts
Cardiac fibroblasts constitute 40%-60% of the heart's cell population and are essential for producing and maintaining the ECM. While fibroblast turnover in a fully developed adult heart is minimal, during MI, fibroblasts differentiate into myofibroblasts in response to inflammatory mediators such as SASP factors including TGF-β1, IL-1, IL-6, and tumor necrosis factor-α (TNF-α)[79,80]. Myofibroblasts play a key role in stabilizing scar tissue by secreting ECM proteins and mediating inflammatory responses, aiding in short-term repair by compensating for the loss of cardiomyocytes. However, the persistence of senescent myofibroblasts leads to maladaptive remodeling and functional decline[81]. For instance, while young mice and rabbit hearts were shown to clear senescent cells post-MI, older rabbit hearts retain these cells longer, driving inflammation, arrhythmias, and progressive cardiac dysfunction[82,83]. This persistence is also observed in elderly patients post-MI, further supporting the role of senescence in age-related arrhythmogenesis[83]. Additionally, fibroblasts play a vital role in modulating hypertrophy by secreting factors such as insulin-like growth factor 1 (IGF1), which promotes collagen synthesis and triggers cardiac hypertrophy, as well as IL-33, which inhibits cardiomyocyte senescence in hypoxic or hypertrophic environment[84,85]. The metabolic state of fibroblasts also significantly influences their function, particularly in aging and remodeling. For example, AMPK activation enhances fibroblast migration and ECM remodeling, while FOXO3A prevents oxidative stress in fibroblasts[86,87]. Furthermore, additional studies suggest that manipulating senescence through SASP factor CCN1 could offer potential therapeutic benefits by promoting repair and reducing fibrosis in the adult mouse heart post-MI[88]. Together, these findings underscore the crucial role of fibroblasts not only in ECM remodeling and scar tissue formation but also in promoting hypertrophy and contributing to age-related arrhythmogenesis.
Immune cells
There is substantial evidence indicating that immune cells play a significant role in CVD initiated by senescence[89]. Indeed, the role of immune cells in the regulation and response to senescence in the heart is very complex. Immune cells, such as neutrophils and macrophages, are known to clear senescent cells and thus can be beneficial in preventing the pathogenesis of CVD induced by senescence[89]. However, during aging and the onset of CVD, immune cells can also become senescent, resulting in enhanced inflammation and driving disease pathogenesis. Senescent macrophages, “foamy macrophages”, have been shown to accumulate in the subendothelial space of arteries and drive plaque formation and atherosclerosis by increasing the expression of SASP factors and proinflammatory cytokines and chemokines. As the plaque advanced, the foamy macrophages increased the expression of MMPs linked to plaque destabilization and rupture, indicating that senescent macrophages are key drivers of plaque formation, maturation, and destabilization[90].
Additionally, like macrophages, T cell senescence plays a critical role in the development of aging-related CVD[91]. It has been shown that T cell senescence enhanced inflammation within plaques by promoting interferon-γ and TNF-α expression. This further exacerbated inflammation, thus promoting the development of atherosclerosis[92,93]. Interestingly, researchers have demonstrated that transferring CD4+ T cells producing interferon-γ from aged mice to young mice triggers an inflammatory response[94]. To validate these findings, it was shown that patients with acute coronary syndromes had a significant increase in senescent CD4+ T cells producing interferon-γ. Moreover, a high number of senescent CD8+ T cells was associated with short-term cardiovascular mortality in acute MI patients[95]. Likewise, patients with hypertension expressed a high frequency of senescent CD8+ T cells, suggesting that they drive hypertension[96]. In an acute liver injury, neutrophils have been shown to transmit ROS when they infiltrate tissue, which induces senescence in neighboring cells by targeting telomeres. Moreover, these effects were accentuated in aging livers, further contributing to organ dysfunction. This study demonstrates how neutrophils are drivers of senescence during chronic inflammation and aging and warrants further research in cardiac-based models[97].
Endothelial cells
Endothelial dysfunction is considered a significant age-related arterial phenotype that plays a key role in CVD among older adults and is mainly caused by endothelial cell senescence[98]. This dysfunction arises from decreased nitric oxide availability due to increased oxidative stress which leads to NF-κB activation, a master regulator of inflammation. Through their SASP, endothelial cells upregulate chemokines and cytokines that recruit and accumulate immune cells in the tissue. In general, the aging endothelium develops a senescent phenotype that promotes oxidative stress, inflammation, and atherosclerosis, potentially leading to MI[99]. Following MI in patients, senescent endothelial cells have been shown to release microparticles that lead to platelet aggregation and thrombogenicity[100]. Interestingly, recent research has demonstrated that older sedentary and not actively exercising adults exhibit greater levels of endothelial cell senescence, which is inversely associated with a clinically relevant assessment of vascular endothelial function. These results clearly demonstrate that endothelial cell senescence may contribute to impaired vascular endothelial function in aging healthy adults[101].
Vascular smooth muscle cells
Vascular smooth muscle cells (VSMCs) work together with endothelial cells to regulate blood pressure, vascular tone, and blood flow. Senescent VSMCs are significant contributors to atherosclerosis development and plaque repair[102]. VSMCs in fibrous caps have been shown to undergo replicative senescence with telomere shortening, which was further accelerated by oxidative stress[103,104]. As VSMCs undergo senescence, they promote the production of proinflammatory SASP mediators, which further drive immune cell chemotaxis[105]. Other than oxidative and inflammatory stress, it was shown that the renin-angiotensin-aldosterone system is a key regulator of VSMC senescence, as enhanced levels of angiotensin II are correlated with VSMC senescence[106].
ASSOCIATION OF AGING, GENDER, SENESCENCE AND CANCER IN CVD
Chronic inflammation serves as a crucial pathophysiological link between CVD and cancer, contributing significantly to atherosclerosis and carcinogenesis. This persistent proinflammatory state, often referred to as "inflammaging", is associated with increased morbidity and mortality in older adults and is largely driven by cellular senescence. The senescent microenvironment plays a critical role in promoting the emergence of heterogeneous cancer stem-like cells, highlighting the complex interplay between aging, senescence, and cancer. Aging leads to cellular senescence, which, while initially acting as a tumor-suppressive mechanism by halting cell proliferation, paradoxically contributes to cancer progression through the SASP. SASP factors induce deleterious inflammation, enhance cell plasticity, and increase the stemness potential in cancer cells, thereby promoting tumorigenesis[107]. Moreover, the release of SASP factors by cancer cells could theoretically induce senescence in neighboring organs, such as the heart, potentially leading to organ-specific toxicities. However, the impact of cancer-induced senescence on adjacent organs, like the heart, has yet to be explored.
Not only does aging lead to senescence, but cancer therapy also causes DNA damage, telomere shortening, an increase in SASP factors, oxidative stress, and mitochondrial dysfunction, which have been shown to lead to cardiac senescence and cardiotoxicity[108]. Although knowledge about various cancer treatments is increasing and cancer survival rates are improving, the incidence of cardiotoxicity leading to CVDs such as cardiomyopathy and HF due to cancer therapy is on the rise[1]. Interestingly, children undergoing cancer treatment are particularly susceptible to developing CVD due to cancer therapy-induced premature senescence, which accelerates the onset of chronic diseases and increases mortality. For instance, a study revealed that roughly 8% of children receiving high cumulative doses of doxorubicin (DOX) or daunorubicin developed significant cardiomyopathy with congestive HF either during therapy or shortly thereafter[109]. Thus, it is becoming increasingly clear that not only is aging a risk factor for the onset of cardiotoxicity associated with senescence, but cancer therapy is also a significant risk factor for premature senescence, consequently affecting cardiovascular health[2].
In addition to age, sex-specific differences in cardiotoxicity, particularly with anthracyclines like DOX, represent a critical factor in heart disease. However, the direct relationship between sex and senescence is not yet fully understood. Studies have shown that female patients, particularly those treated during puberty or adolescence, are more susceptible to chronic anthracycline cardiotoxicity than males[110]. This heightened vulnerability could be due to slower drug clearance in females, resulting in higher anthracycline concentrations in the heart. Hormonal influences may also play a role, as testosterone, a male androgen, has been shown to have a protective effect against DOX-induced cardiac damage. In contrast, estrogen does not appear to offer the same protection. While the exact connection between sex hormones and senescence remains elusive, it has been shown that testosterone inhibits DOX-induced cardiomyocyte senescence, pointing to a potential role of hormonal regulation in senescence pathways[111]. These findings suggest that the observed sex differences in cardiotoxicity could extend to senescence processes, however this area remains under-investigated. Therefore, future studies should focus on exploring the molecular mechanisms linking sex hormones, cardiotoxicity, and senescence to provide more personalized treatments.
CARDIOVASCULAR SENESCENCE IN RESPONSE TO CANCER TREATMENT
While various cancer therapies are associated with cardiotoxic effects, the direct role of cardiac senescence in contributing to this toxicity is still an emerging area of research. One example is the monoclonal antibody trastuzumab, which has been linked to cardiotoxicity; however, its role in triggering cardiac senescence remains less understood[112]. Similarly, treatments like hyperthermia and stem cell transplantation, often used in combination with cancer therapies, may influence cardiovascular health[113,114]. Yet, there is currently a lack of substantial evidence directly connecting these therapies to senescence-driven cardiotoxicity.
In light of these gaps in knowledge, this review will focus on cancer therapies with well-established evidence for inducing cardiovascular senescence and cardiotoxicity, specifically radiotherapy, anthracyclines, and TKIs. Key mechanisms driving cancer therapy-associated cardiotoxicity will be highlighted, as illustrated in Figure 2.
Figure 2. Mechanisms of cardiovascular senescence following cancer treatment with emphasis on doxorubicin. Radiotherapy, anthracyclines [doxorubicin (DOX)] and tyrosine kinase inhibitors (TKIs) are commonly used cancer therapies that impact multiple cell types in the heart, including endothelial cells (ECs), vascular smooth muscle cells (VSMCs), immune cells, fibroblasts, and cardiomyocytes. As a result, different pathways can be activated in response to cancer therapy. (A-D) The main pathways that are activated in response to DOX treatment, although the pathways are also mostly common to other cancer therapies that cause senescence. (A) DNA damage response (DDR) occurs with inhibition of binding of Topoisomerase to DNA, and activates multiple pathways related to the upregulation of p16, ATM/ATR, PI3K, and p38MAPK, which all converge and inhibit cell cycle progression, leading to senescence and release of senescence-associated secretory phenotype (SASP) factors. These are comprised of ILs, cytokines, chemokines, extracellular vesicles, growth factors, bioactive lipids, proteases, and non-coding nucleic acids. (B) Increased production of ROS through several different mechanisms, resulting in mitochondrial dysfunction and SIPS. (C) Telomere dysfunction occurring as a result of decreased expression of TRF2, leading to senescence. (D) Epigenetic changes including DNA methylation, histone modifications, chromatin remodeling and non-coding RNAs trigger and mediate cellular senescence. All these pathways are common to senescent cells and get induced upon cancer therapy, leading to premature senescence in cardiac cells and, as a result, cardiotoxicity. ETC: Electron transport chain; ROS: reactive oxygen species; ER: endoplasmic reticulum; Me: methyl. Created with BioRender.com software.
Radiotherapy
Ionizing radiotherapy (IR) plays a key role in medicine, especially in cancer treatment, in which roughly 50% of patients receive it for curative and/or supportive therapy during their treatment[115,116]. Despite its effectiveness in tumor therapy, this method does not come without side effects. Although the high-energy IR successfully kills cancer cells, it affects the heart and large arteries, resulting in secondary CVDs in cancer survivors. While complications can occur at any dose, at high doses (above 30 Gy/m²), there is a causal association between radiation exposure and CVDs, with a linear increase in the risk of developing CVDs[117]. The Life Span Study of Japanese atomic bomb survivors has shown evidence of an increased risk of CVDs even at moderate (less than 5 Gy) and low doses (much less than 0.5 Gy)[118,119]. Additionally, there are indications of differences in risk between acute and chronic exposure that require further investigation[120]. The first data showing radiation-induced heart disease came from studies of long-term outcomes after IR for breast cancer, thoracic tumors, and Hodgkin’s lymphoma treatment[121]. Different mechanisms of radiation-induced CVD pathogenesis have been reported, such as premature senescence of cardiomyocytes and endothelial cells, inflammation, increased ROS production, mitochondrial dysfunction, and epigenetic modifications. Various downstream molecular pathways contribute to radiation-induced CVDs, which can manifest as atherosclerosis including coronary artery disease and peripheral arterial disease, fibrosis, valvular heart disease, pericarditis, cardiomyopathy and HF, arrhythmias, systemic and pulmonary hypertension, or thromboembolic disease[115,116,121-123]. Aside from promoting cardiomyocyte apoptosis, ionizing radiation causes calcium release from the ER, which affects mitochondrial capacity, leading to calcium overload and subsequent swelling with the release of apoptotic proteins[124]. Premature senescence of endothelial cells is induced by a series of mechanisms such as the activation of ATM/p53/p21 and Akt/PI3K/mTOR; NF-κB, which leads to the secretion of IL-6, a controller of autocrine senescence, telomere shortening accelerated by the oxidative altered state and mitochondrial dysfunction[125]. Chronic vasoconstriction in CVD arises from endothelial nitric oxide synthase (eNOS) uncoupling and nitric oxide (NO) inactivation by ROS. This diminishes vasodilation, which is further compounded by reduced NO production and decreased eNOS activity in senescent endothelial cells[121,122]. Furthermore, mitochondria are extremely sensitive to radiation-induced damage for their reduced repair capacity due to the lack of histones, the high exon/intron ratio, and the presence of the ETC[126]. IR induces ROS production, which directly damages the mitochondrion creating a forward feedback loop, which elevates ROS production. Another mechanism of IR-associated CVDs is represented by the pro-coagulative and pro-thrombotic state generated by stressed and/or damaged endothelial cells. Upon cytokine stimulation, these cells secrete several factors, which, along with low NO availability, contribute to platelet aggregation and thrombus formation[122]. Irradiated endothelial cells also release TGF-β, which activates the fibrotic program of VSMCs[122]. Several studies reported that IR can cause alterations in DNA methylation that can still be observed years after[122]. IR can cause both hypomethylation and hypermethylation of specific genes, with hypermethylation more frequently linked to CVDs[122]. While a relationship between IR and changes in DNA methylation patterns has been established, it remains uncertain whether these changes follow a universal pattern or vary depending on species, model, tissue type, or specific genes. Despite these complexities, evidence suggests that IR can induce both global and gene-specific DNA methylation changes, contributing to the long-term effects on cellular function and potentially promoting diseases like cancer and CVDs[127].
Anthracyclines
Anthracyclines have evolved as one of the most effective and promising treatments for several types of cancers and they are still broadly used to treat leukemia, lymphoma, sarcoma, and breast cancer. However, anthracycline treatment is characterized by potentially severe side effects in various organs, including the heart. Administration of anthracyclines can lead to dose-dependent cardiac toxicity, resulting in left ventricular dysfunction and HF. Several anthracyclines, including DOX, daunorubicin[128], epirubicin[129], and idarubicin[130], have been shown to reduce left ventricular ejection fraction to varying degrees, thereby increasing the risk of congestive HF. DOX, the most prominent compound among anthracyclines, triggers congestive HF in 5.1% of patients with breast and small cell lung cancer, with a predicted rate of 26% in patients with a cumulative dose of equal or above 550 mg/m2. Furthermore, more than 50% of these patients developed a reduction in left ventricular ejection fraction to below 30%[131]. Cardiotoxicity induced by DOX is thought to be mainly caused by myocardial cell apoptosis[132]. However, varying concentrations play a decisive role in the effect of DOX on cell fate. In treated human patients, the range of circulating DOX is typically between 0.025 and 0.250 µmol/L[133], while in in vitro experiments that show an association between DOX and cardiac cell apoptosis, often much higher concentrations of DOX are used (> 1 µmol/L). In rodents, the tolerance for DOX is much higher (0.7 to 2.1 µmol/L)[134]. It was shown that high concentrations (1 µmol/L) of DOX induced neonatal rat cardiomyocyte death, whereas lower concentrations
Master regulatory pathways in DNA damage and senescence
DNA damage can manifest in several forms, such as chemical modifications to nucleotides, single- and double-strand breaks, interstrand crosslinks, and substances directly interfering with DNA[137]. Cells have evolved various mechanisms to limit and confine the DNA damage, including nucleotide and base excision repair, non-homologous end-joining, and homologous recombination, which are employed differentially according to the type of damage and the required accuracy and speed of repair[145]. All these mechanisms are included in a unique cell program defined as the DDR[100,134,138]. Despite the evolution of these repair mechanisms to address DNA damage, mutations continue to occur. The mutation rate was shown to be increased through DOX by DNA interference directly and indirectly. DOX is an intercalating agent that preferentially binds to the GC base pair-rich area and forms adducts, leading to supercoiled DNA, replication stress, and DNA decondensation from nucleosomes[146-148]. DOX’s classic antitumor effect is primarily due to the inhibition of topoisomerase IIb (TOP2b)[149]. This inhibition activates DDR and apoptosis pathways, significantly altering the transcriptome to affect oxidative phosphorylation and mitochondrial biogenesis in cardiomyocytes, leading to cardiotoxicity. Cardiomyocyte-specific deletion of TOP2b was shown to prevent DOX-induced cardiotoxicity in mouse models[149].
DOX-dependent DNA damage can activate not only apoptosis but also cellular senescence[140]. DOX-associated senescence is mediated by activating p38 mitogen-activated protein kinase (MAPK)[142,144]. Once it is activated, it regulates several downstream pathways, including p53, p21 in a p53-independent pathway, p16, and NF-κB[150]. p53 activation promotes cell cycle arrest and is rapidly ubiquitinated and degraded in healthy cells. Once DNA damage occurs, the main proteins that initially take the stage and interact with DNA are ATM and ATR, acting with checkpoint kinase 2 (ChK2) in case of double-strand breaks and checkpoint kinase 1 (ChK1) in case of single-strand breaks, respectively. ATM-Chk2 and ATR-Chk1 stabilize p53 by phosphorylating p53 itself. Phosphorylated p53 can activate apoptosis via p53 upregulated modulator of apoptosis (PUMA) and Phorbol-12-myristate-13-acetate-induced protein 1 (NOXA) or senescence via p21, which in turn inhibits CDK2[134,138]. Activation of NF-κB through p38MAPK leads to the increased secretion of SASP factors (IL-1α, IL-1β, IL-6), antiproliferative proteins (p16, p19, p21, pRB), MMPs, growth factors (TGF-β, GDF15), and MCP1, among others[151].
Another mediator of DOX-associated cellular senescence is p16, mainly referred to as a maintainer of senescence in contrast to p21, which is reported to be an early activator. Through cyclin D-CDK4/6 complex inhibition and RB phosphorylation, p16 leads to RB-E2F complex formation, which in turn inhibits the transcription of cell cycle genes[24,25]. Since DOX treatment has been shown to regulate many inducers of the DDR, including inhibition of TOP2b and mediators such as p38 and p16, it can be assumed that cardiac senescence associated with DOX is partially mediated by the DDR.
Oxidative stress and mitochondrial dysfunction
The heart is particularly vulnerable to oxidative stress, due to high metabolic activity, production of radicals for signaling purposes, and low amounts of antioxidants and antioxidant enzymes[134,152-154]. DOX can cause an increased production of ROS through several different mechanisms. Besides these downstream activated pathways, DOX is a quinone molecule, which is rapidly and easily reduced to its free radical intermediate semiquinone by several enzymes such as NADPH-dependent cytochrome P450 reductases, NADH dehydrogenase (complex I), and cytosolic xanthine oxidase[142]. In the presence of oxygen, semiquinone undergoes redox cycling, leading to the production of ROS[155]. Furthermore, due to its planar structure of the anthracycline ring, DOX acts as an intercalating agent that forms adduct structures with DNA and RNA[142,155]. DOX can bind to nucleic acids regardless of their origin, and its interaction with mtDNA leads to an increase in mutation rate. The mitochondria, having fewer repair mechanisms, cannot compensate over time, resulting in further ROS production mediated by mitochondrial NADPH oxidase[139]. This ultimately leads to general mitochondrial dysfunction and oxidative SIPS[156-159]. The high presence of free radicals and ROS can further cause lipid peroxidation, antioxidant depletion, and diffusion of radicals to the neighboring organelles such as the ER[160]. DOX can also accumulate in the mitochondria, and with its cationic charge, it partially binds to cardiolipin, an anionic phospholipid that is an exclusive constituent of the inner mitochondrial membrane. This interaction results in decreased membrane fluidity and disrupted cristae organization, affecting the proper function of the ETC[134,152]. It has also been shown that DOX increases NF-κB expression both in vitro in cultured cardiomyocytes[161,162] and in vivo[163], leading to oxidative and genotoxic stress, DDR activation[164], p53 upregulation[165], SA-β-gal expression, and SASP production[138,164].
DOX has been reported to affect various cell phenotypes within the heart, including cardiomyocytes, fibroblasts, immune cells, VSMC, and endothelial cells, all of which can undergo DOX-induced senescence. Endothelial cells, in particular, exhibit a unique senescence response to DOX. While they show typical senescence markers such as SA-β-gal positivity and activation of p16 and p21, their secretory response differs from the typical SASP. Specifically, they develop an acute stress-associated phenotype mediated by p38MAPK activation, independent of NF-κB and PI3K/AKT/mTOR pathways[166]. This demonstrates that multiple pathways induced by DOX contribute to the oxidative stress burst, which, in turn, fuels downstream pathways, creating a positive feedback loop that exacerbates cardiac senescence.
Telomere dysfunction
Telomere stability is upheld by various telomere-associated proteins collectively known as the shelterin complex, which prevents telomere ends from being mistaken for double-strand breaks. A crucial protein within this complex, telomeric repeat-binding factor 2 (TRF2), is essential for safeguarding telomeres. Conditional deletion or the overexpression of mutated TRF2 leads to dysfunctional telomeres, telomere fusions, and cell senescence. Telomeres also play a vital role in preventing genome instability, and their impairment through stress stimuli, such as oxidative stress and mitochondrial dysfunction, can lead to replicative senescence[8]. Dysfunctional telomeres mediate senescence via activation of either p53 or p16Ink4a-mediated signaling pathways in human cells or via p53 alone in mouse cells[167-169]. Low doses of DOX treatment resulted in a decreased telomere length[143] and telomerase activity in neonatal rat cardiomyocytes through p53 and MAPK signaling[170]. In human primary VSMCs, low doses of DOX increased the expression of p53 and p21 and decreased the expression of TRF2. This resulted in a reduced preventive potential of TRF2 against DNA damage, leading to telomere dysfunction and senescence[141]. It was shown that both SIPS and expression of mutant TRF2 not only induced senescence by persistent telomere damage in the VSMCs, but also promoted inflammation linked to vascular diseases such as atherosclerosis. Interestingly, DOX-induced senescence through TRF2 modulation was shown to be antagonized by testosterone pre-treatment through PI3K/Akt[111]. Moreover, it was shown that clearance of the senescence cells with senolytics reduced telomere associated foci-positive cells in the aorta and improved vascular phenotypes in aged and hypercholesterolaemic mice[171]. Thus, telomere dysfunction plays a critical role in the onset of senescence and progression of DOX-associated CVDs, and targeting this phenomenon may be a viable therapeutic intervention to improve cardiac health following cancer treatment.
Epigenetic modulation
Epigenetic changes, including chromatin remodeling, histone modification, DNA methylation and non-coding RNAs, regulate genome stability and gene expression and have been shown to trigger and mediate cellular senescence[172]. Increased methylation of the DNA is associated with decreased gene expression and aging[173]. DOX treatment was shown to downregulate DNA methyltransferase 1 and induce hypomethylation in vitro in H9c2 cells and in vivo in rat hearts[156,174]. DNA hypomethylation in late-replicative genes and certain promoter-proximal regions is associated with cell cycle arrest in senescent cells[175]. Histone modifications are closely associated with aging and senescence. During aging, reduced histone levels can alter DNA packaging, thereby influencing gene expression. Notably, DOX has been shown to impair this process by upregulating the level of histone deacetylase in cardiomyocytes[176] and mouse hearts[177]. In recent years, histone deacetylase inhibitors have attracted attention as anti-aging drugs to extend lifespan through the maintenance of cellular histone levels[178]. Epigenetic modulations during aging or DOX treatment affect gene expression and metabolic activities. The decreased availability of substrates such as ATP, NAD+, and acetyl coenzyme A can lead to increased heterochromatin formation[156,179]. In addition, decreased methylation and increased acetylation of peroxisome proliferator-activated receptor gamma coactivator-1 alpha (PGC-1α) through downregulation of histone modifiers, deacetylases, and methyltransferases, reduced the activity of PGC-1α[180]. PGC-1α, a crucial regulator of mitochondrial biogenesis, has been implicated in DOX-induced cardiotoxicity due to disrupted mitochondrial function, which is closely linked to vascular senescence[181]. This impairment can be alleviated by ferruginol, a natural phenol with both antitumor and cardioprotective properties[182]. Although these DOX-induced epigenetic modulations have not yet been directly linked to senescence, these findings suggest that DOX treatment may also influence cardiac senescence by affecting the epigenome, thereby inducing cardiotoxicity.
Tyrosine kinase inhibitors
Molecular targeted agents, particularly TKIs, have emerged as promising candidates for cancer treatment due to the lower expected toxicity compared to conventional cancer drugs. Nevertheless, even FDA-approved TKIs have been shown to induce cardiovascular toxicity[183]. TKI-induced cardiotoxicity is associated with cardiac apoptosis and cell death, which have been shown to be mediated through different mechanisms and cause upregulation of ER stress signaling pathways[184].
Axitinib, a TKI primarily used as a second-line treatment for metastatic renal cell carcinoma, targets vascular endothelial growth factor receptors (VEGFR) 1, 2, and 3[185]. It has been shown to increase ROS production, which contributes to hypercontractility and vascular dysfunction in human VSMCs. This ROS accumulation also induces endothelial senescence in human umbilical vein endothelial cells (HUVECs), as evidenced by elevated
In contrast to that, it was shown that dasatinib (D), a second-generation TKI used to treat chronic myeloid leukemia, attenuated cardiac fibrosis and reduced the expression of the senescence marker p16 in the heart in obese, type 2 diabetic mice, suggesting an inhibitory effect in the senescence signaling pathway[188]. Interestingly, D is currently used as a senolytic drug, especially in combination with quercetin (Q).
Ponatinib, a third-generation TKI used for treating chronic myeloid leukemia, is considered the most cardiotoxic TKI approved by the FDA[189]. It has been linked to increased endothelial senescence in the human aorta, demonstrated by increased SA-β-gal activity upon ponatinib treatment, which was prevented by sodium-glucose cotransporter type 2 inhibitors[190]. Ponatinib-induced endothelial senescence was also detected in HUVECs and was mediated by the Notch-1 signaling pathway[191]. Similar observations were made in mice in vivo, as ponatinib induced cardiac senescence and cardiotoxicity via blocking Notch-1 signaling[192].
Another multi-targeting TKI, sunitinib, was also linked to senescence in several cancer cell lines, including murine breast cancer cells and renal cell carcinoma cells, through the activation of the p53 pathway both
Although sunitinib was not directly linked to cardiovascular senescence yet, it was shown to induce cardiotoxicity in 11% of the investigated patients with imatinib-resistant gastrointestinal stromal tumors[195]. Additionally, left ventricular ejection fraction was < 50% in 20% of the patients, troponin was increased in 18% of the patients, and 47% of the patients developed hypertension. Furthermore, sunitinib induced abnormalities in mouse cardiomyocytes in vivo and apoptosis in cultured rat cardiomyocytes[195]. Therefore, it would be crucial to investigate whether sunitinib also induces senescence in the heart to establish possible treatment options using senotherapies.
The epidermal growth factor receptor (EGFR) inhibitor gefitinib is another TKI associated with cardiotoxicity and senescence. Gefitinib is normally used to treat non-small cell lung cancers (NSCLCs). In various NSCLC cell lines, high doses of gefitinib induced apoptosis, while lower, clinically relevant doses of gefitinib induced premature senescence. This was accompanied by an increase in p16INK4A, p21WAF1/Cip1 and p27Kip1 levels causing G1 cell cycle arrest[196]. Gefitinib was shown to cause cardiotoxicity in rats, which was attenuated by pre-treatment with the glucagon-like peptide 1 receptor agonist liraglutide, normally used to lower blood sugar levels[196]. Interestingly, liraglutide demonstrated antioxidant properties by decreasing NF-κB and activating survival pathways such as the MAPK and AKT pathways. As NF-κB is an important regulator of aging, through its roles in cellular senescence and inflammatory pathways, it would be relevant to investigate the role of senescence in gefitinib-induced cardiotoxicity[196]. Further research on the molecular mechanisms of TKI-induced cardiotoxicity is needed to unravel the mechanisms by which targeted therapies also induce CVDs, and to develop more personalized senotherapeutics for TKI-induced cardiotoxicity.
SENOTHERAPEUTICS FOR TARGETING SENESCENT CELLS
As cancer therapeutics lead to cardiovascular senescence, targeting senescent cells is a promising strategy to alleviate senescence-induced CVD. Senescent cells have an active DDR, increased mitochondrial dysfunction and membrane permeability, increased ROS production, and heightened metabolic flux, and produce an inflammatory SASP, creating hostile internal and external microenvironments[197]. Despite this, senescent cells remain viable and accumulate in tissues with age, partly due to increased survival and resistance to apoptosis. Thus, senotherapies that target senescent cells are being explored, such as senolytics and senomorphics. Senolytics induce apoptosis specifically in senescent cells without affecting non-senescent cells by targeting the survival pathways including B-cell lymphoma (BCL)-2 family members, p53/p21Cip, ephrins (EFNB1 or 3), PI3K, and PAI-1 and 2[198]. On the other hand, senomorphics prevent the development of the SASP, thereby decreasing the harmful effects of senescent cells on their environment without necessarily eliminating the cells. Various senotherapeutics and their mechanisms are also illustrated in Figure 3.
Figure 3. Senotherapeutics for targeting cardiovascular senescence. The circular figure presents senolytics, which induce apoptosis specifically in senescent cells by targeting several survival pathways, and senomorphics, which prevent the development of the SASP, restoring the cell to a less agitated state. The molecules affected by each drug are presented in the figure and indicate the pathways that are targeted in senescent cells. Created with BioRender.com software.
Senolytics
Navitoclax (ABT263) is a BH3-only protein mimetic that acts as a BCL-2 inhibitor. With its high affinity for pro-survival BCL-2 family proteins such as BCL-XL, BCL-2, and BCL-W, it effectively induces apoptosis in senescent cells[199]. Senolytic therapies that target tumor cells are heavily studied because tumor cells generally also become senescent, and the success of anticancer treatments often hinges on the ability to induce cancer cell death by apoptosis[200]. Navitoclax has been investigated in many preclinical studies and clinical trials for eliminating senescent cells[201,202]. The most recent phase 2 clinical trial involving both navitoclax and ruxolitinib was performed by AbbVie for the treatment of myelofibrosis, a rare and difficult-to-treat blood cancer[203]. The findings of the trial showed symptomatic improvement in the cohort of patients; however, the treatment led to adverse events in 97% of patients, with thrombocytopenia, anemia, and neutropenia being the most prominent effects[203]. While it is known for its use in the direct targeting of tumor senescent cells, its role in targeting cardiac senescent cells is less studied. Studies have shown that navitoclax reduced cardiotoxicity from treatments such as DOX by lowering senescence markers and restoring cardiac function in mice[204]. Further studies in cardiac models are required to further establish its therapeutic benefit for eliminating cardiac senescence induced by cancer treatments. Moreover, it has been shown that the two selective BCL-XL inhibitors A1331852 and A1155463 are also effective in clearing senescent cells in vitro[205]. As these agents do not target BCL-2 like navitoclax, they are believed to reduce BCL-2-induced neutrophil toxicity[200]. However, these agents will need to be tested in preclinical models to establish their senolytic and adverse effects. As such, there is a need for novel and improved senotherapeutic drugs and combinatorial approaches to safely eliminate senescent cells from multiple organs or even within a single tissue.
Dasatinib (D) and quercetin (Q) are TKIs that act as senolytics. D is known to interfere with EFNB-dependent suppression of apoptosis and was shown to selectively reduce the viability of senescent human preadipocytes, but it is less effective on senescent HUVECs. In contrast, Q, a natural flavonoid that inhibits PI3K, selectively reduces the viability of senescent HUVECs more than proliferating cells but is less effective on preadipocytes[206]. Studies showed that a combination of D and Q selectively targets both senescent preadipocytes and endothelial cells more effectively than either agent alone[206]. The combination was tested in a phase I clinical trial to target cellular senescence in Alzheimer’s disease, with the results demonstrating the safety and tolerability of D and Q in older adults[207]. The next clinical trials will assess the safety, feasibility, and efficacy of the drug combination for targeting cellular senescence in patients[208].
Fisetin, another member of the flavonoid family, targets multiple signaling pathways, including PI3K/AKT, NF-κB, p38MAPK, and BCL-2/BCL-XL[205]. In vivo, fisetin effectively reduced cell cycle inhibitor p16Ink4a-expressing cells, including T lymphocytes, natural killer, and endothelial cells, particularly in the subcutaneous fat of aged mice. It also extended the health and lifespan of older animals, with no adverse side effects even when used at high doses[209]. In vitro, fisetin was shown to inhibit the activity of several proinflammatory cytokines secreted by SASP, including TNFα, IL-6, and the transcription factor NF-κB[210]. Compared to other flavonoids, fisetin was the most potent in reducing senescence markers and SASP in multiple cell types both in in vitro models including primary murine embryonic fibroblasts or human fibroblasts, and in vivo in aged mice and sheep[209,211]. Thus, given fisetin’s presence in common foods, availability as a dietary supplement, and lack of reported adverse side effects, it holds promise for human therapies, with phase II clinical trials currently testing whether fisetin improves physical function and frailty in women who received chemotherapy for breast cancer[212,213].
Targeting SASP with senomorphics
Senomorphic therapies, which aim to reduce or modify the synthesis of molecules contributing to SASP, thereby suppressing the proinflammatory properties of senescent cells, are being explored as treatments to mitigate cellular senescence. Senomorphic substances regulate biochemical pathways such as mTOR, p38-MAPK, NF-κB, Janus kinases (JAK)/signal transducer and activator of transcription proteins (STAT), and glucocorticoid receptors[214]. Rapamycin, an FDA-approved anticancer drug, is an mTOR inhibitor that has been shown to exert senomorphic properties by reducing senescence markers p16 and p21 in an Nrf2-dependent manner. It has been shown to selectively inhibit the secretion of SASP components and reduce the activation of the STAT3 pathway without affecting cell cycle arrest, both in vitro in human cells and
Non-coding RNAs as senomorphics
Senomorphic compounds such as non-coding RNAs including micro RNAs (miRNAs) and long non-coding RNAs (lncRNAs) can offer additional avenues for combatting cardiac senescence as they are known to affect SASP expression and interfere with cell cycle pathways. Previous research has demonstrated that extracellular miRNAs are part of the SASP, with miRNAs from senescent cells potentially playing a significant role in disrupting tissue homeostasis during aging[227]. The primary difference between miRNAs and lncRNAs is that miRNAs are small, non-coding RNAs that regulate gene expression post-transcriptionally by binding to messenger RNAs, whereas lncRNAs are longer non-coding RNAs that can regulate gene expression through diverse mechanisms, including acting as scaffolds, decoys, or guides for protein complexes and influencing chromatin structure[228]. While many non-coding RNAs have been studied for their senotherapeutic potential, their benefits in reducing cardiovascular toxicity induced by senescence have not been studied[227]. However, some miRNAs have been linked to cardiovascular aging and could be considered as potential therapies for targeting cardiac senescence induced by cancer treatments. For example, miR-34a, a key regulator of p66Shc[214], is promising as a therapeutic target, as the knockdown of p66Shc has been shown to prevent senescence[229] and extend the lifespan of the animals by 30%[230], as well as inhibit the formation of plaque[231]. miR-34a targets the pro-survival gene SIRT1, leading to a positive feedback loop with the acetylation of p53 that promotes apoptosis through increased levels of pro-apoptotic proteins[232]. Interestingly, upregulated miR-34a expression was implicated in inducing cardiac senescence in patients with type 2 diabetes by decreasing SIRT1 expression. Thus, inhibition of miR-34a was shown to reduce deleterious effects in cardiomyocytes under high glucose conditions, suggesting potential benefits for reducing cardiac senescence[233]. miR-17-3p is another miRNA that was shown to play a role in cardiac senescence and aging. Studies demonstrated that aged mouse hearts downregulated miR-17-3p expression, leading to an increase in its target protein prostate apoptosis response-4 (PAR-4)[234]. PAR-4 is a pro-apoptotic protein that, when expressed, downregulates the expression of the anti-apoptotic proteins CCAAT/enhancer-binding protein beta (CEBPB) and focal adhesion kinase (FAK), and has been shown to contribute to enhanced cellular senescence and apoptosis in mouse cardiac fibroblast cells[235]. As such, miR-17-3p could be a target for cardiac senescence following cancer treatment in patients, but further research in appropriate preclinical cancer models is required to validate the safety and potential efficacy of the treatment. Lastly, an interesting miRNA to point out is miR-22, as it has been identified to have a role in cardiac senescence and apoptosis[236]. miR-22 targets osteoglycin, which was shown to have a cardioprotective effect by reducing collagen production and fibrosis. Researchers show that osteoglycin is downregulated with age, along with an upregulation of miR-22 expression in old mice models[236,237]. Moreover, patients with MI also showed an upregulation of circulating miR-22 expression levels, correlating with increased fibrosis in the heart[238]. This suggests a possibility of using miR-22 as a potential biomarker and therapeutic target for cardiac senescence and fibrosis as an outcome of cardiotoxicity. Furthermore, in a recent study, researchers explored novel miRNAs that could be used as biomarkers for senescence. This study provided evidence that miRNA expression patterns correlated with the increase of senescent cells in the tissue, and proposed a selection of senomiRs as minimally invasive biomarkers of senescent cell load[239]. Overall, the field of miRNAs and their use for therapeutic intervention of senescence is still in its infancy; however, these recent studies provide the basis for future investigation.
Unlike miRNAs that repress the translation of mRNA, lncRNAs can upregulate or downregulate gene expression and have been implicated in senescence, with a few recent studies correlating their expression with cardiac aging[228]. Interestingly, studies showed that the downregulation of lncRNA MALAT1 correlated with increased expression of miR-34a levels in aged mice. MALAT1 expression was shown to control cell cycle progression, and its depletion was shown to lead to activation of p53 and its target genes. Moreover, MALAT1-depleted cells showed enhanced SA-β-gal staining indicative of cellular senescence and a change in cellular morphology to a flat-shaped cell[240]. Thus, it is very likely that MALAT1 is downregulated, along with an upregulation of miR-34a, following cancer treatments that induce cardiac senescence and cardiotoxicity. However, the role of MALAT1 in cardiac senescence needs to be further investigated. Overall, the study of lncRNAs in the progression of CVDs is relatively recent and they are not as thoroughly investigated as miRNAs[241]. Unlike miRNAs, lncRNAs are not highly conserved, and since most research is conducted on mouse models or in vitro, it remains uncertain whether lncRNAs will function similarly in humans[242].
Other approaches to target senescent cells
Additionally, various other senolytic therapies have been explored for targeting cardiac senescence. One example is a vaccine against glycoprotein nonmetastatic melanoma protein B (GPNMB), which is typically enriched in senescent cells, as well as in vascular endothelial cells and leukocytes in both mice and humans with atherosclerosis. This vaccine has been shown to reduce the atherosclerotic burden in apolipoprotein E knockout mice fed a high-fat diet[243]. Furthermore, senescent cell accumulation in obese and atherosclerotic mice was reduced by the inhibition of glutaminase 1 through BPTES [bis-2-(5-phenylacetamido-1,2,4-thiadiazol-2-yl) ethyl sulfide]. Inhibition of glutaminase 1 inhibits the ammonia production induced by glutaminolysis, which would otherwise improve the survival of the senescent cells. Therefore, glutaminolysis serves as an interesting target for further senolytics[244]. Another interesting class of drugs are cardiac glycosides, including digoxin. Digoxin inhibits the Na/K-ATPase pump, which unbalances the electrochemical gradient and is normally used to treat HF and atrial fibrillation, but it also has senolytic potential[245,246]. Senescent cells are particularly susceptible to this, as they display a more polarized state than healthy cells[246]. Moreover, in a mouse model of lung fibrosis induced by the administration of senescent human fibroblasts directly into the lungs, in vivo treatment with digoxin preferentially triggered cell death in senescent cells and significantly reduced the extent of fibrosis[246]. Thus, digoxin could serve as a promising therapeutic intervention to target cardiac senescence. Another example of a senolytic intervention is the use of chimeric antigen receptor T (CAR-T) cells, whereby due to the immunogenic activity of senescent cells, senescent cell-specific antigens can be targeted. It was shown that CAR-T cells specific for the urokinase-type plasminogen activator receptor (uPAR), a cell-surface protein induced during senescence, ablated senescent cells in vitro and in vivo and reduced liver fibrosis, as well as extending the lifespan of mice[247]. Thus, this approach could be promising for targeting cardiac senescence induced by cancer treatments. However, further research in a cancer model is required to test the safety of an immunotherapy that targets senescence in a host already affected by cancer.
FUTURE DIRECTIONS AND CONCLUSION
Future research in the field of senotherapeutics should focus on refining existing therapies and developing novel drugs to effectively target senescent cells and mitigate their adverse effects on cardiovascular health, particularly in cancer patients. While most of the senotherapies discussed have not been investigated for alleviating the cardiotoxic effects of cancer drugs, navitoclax is the only senolytic that has been tested in preclinical trials for this purpose. However, navitoclax and selective BCL-XL inhibitors like A1331852 and A1155463, while effective, have been shown to have serious side effects, including thrombocytopenia and neutropenia, which are frequently present in cancer patients due to cancer therapy or hemato-oncological disease itself. This raises an important question: at what cost do we treat one disease before potentially causing another? Is there a drug with no side effects that can efficiently and safely mitigate cardiotoxicity without causing other diseases? Among the promising candidates, fisetin has demonstrated significant potential in reducing senescence markers and extending lifespan without major side effects. Given fisetin's presence in common foods, availability as a dietary supplement, and lack of reported adverse side effects, it holds promise for use in patients undergoing cancer therapies, especially young adults who are more prone to develop premature senescence. Ongoing clinical trials testing fisetin’s impact on physical function and frailty in chemotherapy-treated women may confirm its viability as a therapeutic option for reducing cardiotoxicity[212]. Similarly, the combination of D and Q has shown efficacy in preclinical models and early clinical trials for Alzheimer’s disease, suggesting that further trials targeting CVDs in cancer patients could yield positive results[207]. The role of non-coding RNAs, particularly miR-34a and miR-17-3p, in cardiac senescence requires further investigation, with a focus on developing cell-specific delivery systems to minimize off-target effects. Long non-coding RNAs like MALAT1 also hold therapeutic potential, and their mechanisms and interactions with miRNAs should be explored in greater depth. Senomorphic approaches, including SASP inhibitors like rapamycin and metformin, have shown promise, but their side effects necessitate the search for novel compounds or combination therapies that offer similar benefits without adverse reactions. Novel SASP inhibitors such as SR12343 and JAK/STAT pathway inhibitors like ruxolitinib should be further validated in both preclinical and clinical settings for their potential to target senescent cells, particularly cardiac senescence post-cancer treatment. Immunotherapies, including CAR-T cells targeting senescent cell-specific antigens and vaccines against proteins like GPNMB, present innovative solutions for selectively eliminating senescent cells. These strategies warrant further exploration, particularly in addressing CVDs and cancer therapy-induced cardiotoxicity. A major concern in cancer patients are drug interactions. Administering an anti-inflammatory agent could inadvertently nourish the tumor environment. Thus, the use of anti-inflammatory therapeutics may not be a safe option for patients undergoing cancer treatment or in remission.
In conclusion, the accumulation of senescent cells due to cancer therapies poses a significant challenge in the progression of CVDs. While current senolytic and senomorphic therapies show promise, their clinical application requires careful balancing of efficacy and safety to avoid adverse effects. Future research should focus on developing targeted delivery mechanisms, particularly for non-coding RNA therapies, and refining existing senolytics to minimize off-target effects. Through continued exploration and clinical validation, the development of innovative therapies can ultimately improve the management of cardiotoxic side effects, enhancing both cardiovascular health and overall patient outcomes. A comprehensive understanding and targeted intervention in senescence pathways hold the key to mitigating the adverse effects of cancer treatments and promoting healthier aging. Additionally, a more targeted release of therapies, rather than a systemic delivery, could help localize the treatment and reduce systemic side effects, further optimizing patient care.
DECLARATIONS
Authors’ contributions
Conceptualized the review: Grüterich V, Kuster GM
Wrote the manuscript and prepared the final version: Alshoubaki YK, Grüterich V
Made the figures: Alshoubaki YK, Grüterich V, Zollet V
Guided the writing and edited the manuscript: Alshoubaki YK, Kuster GM
Availability of data and materials
Not applicable.
Financial support and sponsorship
This work was funded by the Swiss National Science Foundation (SNSF; grant number 219250 to Kuster GM). All figures were created with BioRender software (www.biorender.com), agreement numbers NE27D55IWC, AK27D4M7N6, TX27D4LNYC, and ZY27D4LT82.
Conflicts of interest
Kuster GM received speaker and consultant fees from Janssen and consultant fees from PAGE Therapeutics, all paid to the institution and unrelated to this work. While the other authors declared that there are no conflicts of interest.
Ethical approval and consent to participate
Not applicable.
Consent for publication
Not applicable.
Copyright
© The Author(s) 2024.
REFERENCES
1. Sturgeon KM, Deng L, Bluethmann SM, et al. A population-based study of cardiovascular disease mortality risk in US cancer patients. Eur Heart J 2019;40:3889-97.
2. Curigliano G, Lenihan D, Fradley M, et al. Management of cardiac disease in cancer patients throughout oncological treatment: ESMO consensus recommendations. Ann Oncol 2020;31:171-90.
3. Nagy A, Börzsei D, Hoffmann A, et al. A comprehensive overview on chemotherapy-induced cardiotoxicity: insights into the underlying inflammatory and oxidative mechanisms. Cardiovasc Drugs Ther 2024.
4. Evangelou K, Vasileiou PVS, Papaspyropoulos A, et al. Cellular senescence and cardiovascular diseases: moving to the "heart" of the problem. Physiol Rev 2023;103:609-47.
6. Yang Y, Mihajlovic M, Masereeuw R. Protein-bound uremic toxins in senescence and kidney fibrosis. Biomedicines 2023;11:2408.
7. Hayflick L, Moorhead PS. The serial cultivation of human diploid cell strains. Exp Cell Res 1961;25:585-621.
8. Rossiello F, Jurk D, Passos JF, d'Adda di Fagagna F. Telomere dysfunction in ageing and age-related diseases. Nat Cell Biol 2022;24:135-47.
10. Li D, Li Y, Ding H, Wang Y, Xie Y, Zhang X. Cellular senescence in cardiovascular diseases: from pathogenesis to therapeutic challenges. J Cardiovasc Dev Dis 2023;10:439.
11. Serrano M, Lin AW, McCurrach ME, Beach D, Lowe SW. Oncogenic ras provokes premature cell senescence associated with accumulation of p53 and p16INK4a. Cell 1997;88:593-602.
12. Liu XL, Ding J, Meng LH. Oncogene-induced senescence: a double edged sword in cancer. Acta Pharmacol Sin 2018;39:1553-8.
13. Freund A, Laberge RM, Demaria M, Campisi J. Lamin B1 loss is a senescence-associated biomarker. Mol Biol Cell 2012;23:2066-75.
14. Shimi T, Butin-Israeli V, Adam SA, et al. The role of nuclear lamin B1 in cell proliferation and senescence. Genes Dev 2011;25:2579-93.
15. Miller KN, Dasgupta N, Liu T, Adams PD, Vizioli MG. Cytoplasmic chromatin fragments-from mechanisms to therapeutic potential. Elife 2021;10:e63728.
16. Prokocimer M, Davidovich M, Nissim-Rafinia M, et al. Nuclear lamins: key regulators of nuclear structure and activities. J Cell Mol Med 2009;13:1059-85.
17. Sadaie M, Salama R, Carroll T, et al. Redistribution of the Lamin B1 genomic binding profile affects rearrangement of heterochromatic domains and SAHF formation during senescence. Genes Dev 2013;27:1800-8.
18. Narita M, Nũnez S, Heard E, et al. Rb-mediated heterochromatin formation and silencing of E2F target genes during cellular senescence. Cell 2003;113:703-16.
19. Ivanov A, Pawlikowski J, Manoharan I, et al. Lysosome-mediated processing of chromatin in senescence. J Cell Biol 2013;202:129-43.
20. Takahashi A, Imai Y, Yamakoshi K, et al. DNA damage signaling triggers degradation of histone methyltransferases through APC/CCdh1 in senescent cells. Mol Cell 2012;45:123-31.
21. Zou L. Single- and double-stranded DNA: building a trigger of ATR-mediated DNA damage response. Genes Dev 2007;21:879-85.
22. Xie A, Hartlerode A, Stucki M, et al. Distinct roles of chromatin-associated proteins MDC1 and 53BP1 in mammalian double-strand break repair. Mol Cell 2007;28:1045-57.
23. Bartkova J, Horejsí Z, Sehested M, et al. DNA damage response mediators MDC1 and 53BP1: constitutive activation and aberrant loss in breast and lung cancer, but not in testicular germ cell tumours. Oncogene 2007;26:7414-22.
24. Huang W, Hickson LJ, Eirin A, Kirkland JL, Lerman LO. Cellular senescence: the good, the bad and the unknown. Nat Rev Nephrol 2022;18:611-27.
25. Safwan-Zaiter H, Wagner N, Wagner KD. P16INK4A-more than a senescence marker. Life 2022;12:1332.
26. Passos JF, Saretzki G, Ahmed S, et al. Mitochondrial dysfunction accounts for the stochastic heterogeneity in telomere-dependent senescence. PLoS Biol 2007;5:e110.
27. Passos JF, Nelson G, Wang C, et al. Feedback between p21 and reactive oxygen production is necessary for cell senescence. Mol Syst Biol 2010;6:347.
28. Nelson G, Kucheryavenko O, Wordsworth J, von Zglinicki T. The senescent bystander effect is caused by ROS-activated NF-κB signalling. Mech Ageing Dev 2018;170:30-6.
29. Di Micco R, Fumagalli M, Cicalese A, et al. Oncogene-induced senescence is a DNA damage response triggered by DNA hyper-replication. Nature 2006;444:638-42.
30. Kwon SM, Hong SM, Lee YK, Min S, Yoon G. Metabolic features and regulation in cell senescence. BMB Rep 2019;52:5-12.
31. Miwa S, Kashyap S, Chini E, von Zglinicki T. Mitochondrial dysfunction in cell senescence and aging. J Clin Invest 2022;132:e158447.
32. Wu S, Zou MH. AMPK, mitochondrial function, and cardiovascular disease. Int J Mol Sci 2020;21:4987.
33. Tang X, Chen XF, Wang NY, et al. SIRT2 acts as a cardioprotective deacetylase in pathological cardiac hypertrophy. Circulation 2017;136:2051-67.
34. Wiley CD, Campisi J. The metabolic roots of senescence: mechanisms and opportunities for intervention. Nat Metab 2021;3:1290-301.
35. Wiley CD, Campisi J. From ancient pathways to aging cells-connecting metabolism and cellular senescence. Cell Metab 2016;23:1013-21.
36. Kwon Y, Kim JW, Jeoung JA, Kim MS, Kang C. Autophagy is pro-senescence when seen in close-up, but anti-senescence in long-shot. Mol Cells 2017;40:607-12.
37. Lee BY, Han JA, Im JS, et al. Senescence-associated β-galactosidase is lysosomal β-galactosidase. Aging Cell 2006;5:187-95.
38. Di Micco R, Krizhanovsky V, Baker D, d'Adda di Fagagna F. Cellular senescence in ageing: from mechanisms to therapeutic opportunities. Nat Rev Mol Cell Biol 2021;22:75-95.
39. Wiley CD, Sharma R, Davis SS, et al. Oxylipin biosynthesis reinforces cellular senescence and allows detection of senolysis. Cell Metab 2021;33:1124-36.e5.
40. Takasugi M, Okada R, Takahashi A, Virya Chen D, Watanabe S, Hara E. Small extracellular vesicles secreted from senescent cells promote cancer cell proliferation through EphA2. Nat Commun 2017;8:15729.
41. Weiner-Gorzel K, Dempsey E, Milewska M, et al. Overexpression of the microRNA miR-433 promotes resistance to paclitaxel through the induction of cellular senescence in ovarian cancer cells. Cancer Med 2015;4:745-58.
42. Borghesan M, Fafián-Labora J, Eleftheriadou O, et al. Small extracellular vesicles are key regulators of non-cell autonomous intercellular communication in senescence via the interferon protein IFITM3. Cell Rep 2019;27:3956-71.e6.
43. Sagini K, Urbanelli L, Costanzi E, et al. Oncogenic H-ras expression induces fatty acid profile changes in human fibroblasts and extracellular vesicles. Int J Mol Sci 2018;19:3515.
44. Jacob J, Aggarwal A, Aggarwal A, et al. Senescent chondrogenic progenitor cells derived from articular cartilage of knee osteoarthritis patients contributes to senescence-associated secretory phenotype via release of IL-6 and IL-8. Acta Histochem 2022;124:151867.
45. Kuilman T, Michaloglou C, Vredeveld LCW, et al. Oncogene-induced senescence relayed by an interleukin-dependent inflammatory network. Cell 2008;133:1019-31.
46. Laberge RM, Sun Y, Orjalo AV, et al. MTOR regulates the pro-tumorigenic senescence-associated secretory phenotype by promoting IL1A translation. Nat Cell Biol 2015;17:1049-61.
47. Nakamura Y, Aihara R, Iwata H, Kuwayama T, Shirasuna K. IL1B triggers inflammatory cytokine production in bovine oviduct epithelial cells and induces neutrophil accumulation via CCL2. Am J Reprod Immunol 2021;85:e13365.
48. Orjalo AV, Bhaumik D, Gengler BK, Scott GK, Campisi J. Cell surface-bound IL-1α is an upstream regulator of the senescence-associated IL-6/IL-8 cytokine network. Proc Natl Acad Sci USA 2009;106:17031-6.
49. Ortiz-Montero P, Londoño-Vallejo A, Vernot JP. Senescence-associated IL-6 and IL-8 cytokines induce a self- and cross-reinforced senescence/inflammatory milieu strengthening tumorigenic capabilities in the MCF-7 breast cancer cell line. Cell Commun Signal 2017;15:17.
50. Rossi M, Anerillas C, Idda ML, et al. Pleiotropic effects of BAFF on the senescence-associated secretome and growth arrest. Elife 2023;12:e84238.
51. Wang AP, Yang F, Tian Y, et al. Pulmonary artery smooth muscle cell senescence promotes the proliferation of PASMCs by paracrine IL-6 in hypoxia-induced pulmonary hypertension. Front Physiol 2021;12:656139.
52. Yamagishi R, Kamachi F, Nakamura M, et al. Gasdermin D-mediated release of IL-33 from senescent hepatic stellate cells promotes obesity-associated hepatocellular carcinoma. Sci Immunol 2022;7:eabl7209.
53. Zhou J, Chen H, Wang Q, et al. Sirt1 overexpression improves senescence-associated pulmonary fibrosis induced by vitamin D deficiency through downregulating IL-11 transcription. Aging Cell 2022;21:e13680.
54. Coppé JP, Patil CK, Rodier F, et al. Senescence-associated secretory phenotypes reveal cell-nonautonomous functions of oncogenic RAS and the p53 tumor suppressor. PLoS Biol 2008;6:2853-68.
55. Chambers ES, Vukmanovic-Stejic M, Shih BB, et al. Recruitment of inflammatory monocytes by senescent fibroblasts inhibits antigen-specific tissue immunity during human aging. Nat Aging 2021;1:101-13.
56. Cheng N, Kim KH, Lau LF. Senescent hepatic stellate cells promote liver regeneration through IL-6 and ligands of CXCR2. JCI Insight 2022;7:e158207.
57. Hwang HJ, Lee YR, Kang D, et al. Endothelial cells under therapy-induced senescence secrete CXCL11, which increases aggressiveness of breast cancer cells. Cancer Lett 2020;490:100-10.
58. Jin HJ, Lee HJ, Heo J, et al. Senescence-associated MCP-1 secretion is dependent on a decline in BMI1 in human mesenchymal stromal cells. Antioxid Redox Signal 2016;24:471-85.
59. Kawagoe Y, Kawashima I, Sato Y, Okamoto N, Matsubara K, Kawamura K. CXCL5-CXCR2 signaling is a senescence-associated secretory phenotype in preimplantation embryos. Aging Cell 2020;19:e13240.
60. Shen L, Chen Y, Cheng J, et al. CCL5 secreted by senescent theca-interstitial cells inhibits preantral follicular development via granulosa cellular apoptosis. J Cell Physiol 2019;234:22554-64.
61. Sturmlechner I, Zhang C, Sine CC, et al. p21 produces a bioactive secretome that places stressed cells under immunosurveillance. Science 2021;374:eabb3420.
62. Takikawa T, Hamada S, Matsumoto R, et al. Senescent human pancreatic stellate cells secrete CXCR2 agonist CXCLs to promote proliferation and migration of human pancreatic cancer AsPC-1 and MIAPaCa-2 cell lines. Int J Mol Sci 2022;23:9275.
63. Zang J, Ye J, Zhang C, Sha M, Gao J. Senescent hepatocytes enhance natural killer cell activity via the CXCL-10/CXCR3 axis. Exp Ther Med 2019;18:3845-52.
64. Zheng X, Wang Q, Xie Z, Li J. The elevated level of IL-1α in the bone marrow of aged mice leads to MSC senescence partly by down-regulating Bmi-1. Exp Gerontol 2021;148:111313.
65. Kim H, Jang J, Song MJ, et al. Inhibition of matrix metalloproteinase expression by selective clearing of senescent dermal fibroblasts attenuates ultraviolet-induced photoaging. Biomed Pharmacother 2022;150:113034.
66. Eren M, Boe AE, Murphy SB, et al. PAI-1-regulated extracellular proteolysis governs senescence and survival in Klotho mice. Proc Natl Acad Sci USA 2014;111:7090-5.
67. Özcan S, Alessio N, Acar MBB, et al. Unbiased analysis of senescence associated secretory phenotype (SASP) to identify common components following different genotoxic stresses. Aging 2016;8:1316-27.
68. Mehdizadeh M, Aguilar M, Thorin E, Ferbeyre G, Nattel S. The role of cellular senescence in cardiac disease: basic biology and clinical relevance. Nat Rev Cardiol 2022;19:250-64.
69. Basisty N, Kale A, Jeon OH, et al. A proteomic atlas of senescence-associated secretomes for aging biomarker development. PLoS Biol 2020;18:e3000599.
70. Wang B, Han J, Elisseeff JH, Demaria M. The senescence-associated secretory phenotype and its physiological and pathological implications. Nat Rev Mol Cell Biol 2024;25:958-78.
71. Ohtani N. The roles and mechanisms of senescence-associated secretory phenotype (SASP): can it be controlled by senolysis? Inflamm Regen 2022;42:11.
72. Zhai P, Sadoshima J. Cardiomyocyte senescence and the potential therapeutic role of senolytics in the heart. J Cardiovasc Aging 2024;4:18.
73. Luan Y, Zhu X, Jiao Y, et al. Cardiac cell senescence: molecular mechanisms, key proteins and therapeutic targets. Cell Death Discov 2024;10:78.
74. von Zglinicki T, Wan T, Miwa S. Senescence in post-mitotic cells: a driver of aging? Antioxid Redox Signal 2021;34:308-23.
75. Anderson R, Lagnado A, Maggiorani D, et al. Length-independent telomere damage drives post-mitotic cardiomyocyte senescence. EMBO J 2019;38:e100492.
76. Shan H, Li T, Zhang L, et al. Heme oxygenase-1 prevents heart against myocardial infarction by attenuating ischemic injury-induced cardiomyocytes senescence. EBioMedicine 2019;39:59-68.
77. Suda M, Paul KH, Minamino T, et al. Senescent cells: a therapeutic target in cardiovascular diseases. Cells 2023;12:1296.
78. Turdi S, Fan X, Li J, et al. AMP-activated protein kinase deficiency exacerbates aging-induced myocardial contractile dysfunction. Aging Cell 2010;9:592-606.
79. Gorski DJ, Petz A, Reichert C, Twarock S, Grandoch M, Fischer JW. Cardiac fibroblast activation and hyaluronan synthesis in response to hyperglycemia and diet-induced insulin resistance. Sci Rep 2019;9:1827.
80. Wang L, Jiang P, He Y, et al. A novel mechanism of Smads/miR-675/TGFβR1 axis modulating the proliferation and remodeling of mouse cardiac fibroblasts. J Cell Physiol 2019;234:20275-85.
81. Czubryt MP. Common threads in cardiac fibrosis, infarct scar formation, and wound healing. Fibrogenesis Tissue Repair 2012;5:19.
82. Shibamoto M, Higo T, Naito AT, et al. Activation of DNA damage response and cellular senescence in cardiac fibroblasts limit cardiac fibrosis after myocardial infarction. Int Heart J 2019;60:944-57.
83. Baggett BC, Murphy KR, Sengun E, et al. Myofibroblast senescence promotes arrhythmogenic remodeling in the aged infarcted rabbit heart. Elife 2023;12:e84088.
84. Ock S, Ham W, Kang CW, Kang H, Lee WS, Kim J. IGF-1 protects against angiotensin II-induced cardiac fibrosis by targeting
85. Tao A, Song J, Lan T, et al. Cardiomyocyte-fibroblast interaction contributes to diabetic cardiomyopathy in mice: role of HMGB1/TLR4/IL-33 axis. Biochim Biophys Acta 2015;1852:2075-85.
86. Qi H, Liu Y, Li S, et al. Activation of AMPK attenuated cardiac fibrosis by inhibiting CDK2 via p21/p27 and miR-29 family pathways in rats. Mol Ther Nucleic Acids 2017;8:277-90.
87. Chiribau CB, Cheng L, Cucoranu IC, Yu YS, Clempus RE, Sorescu D. FOXO3A regulates peroxiredoxin III expression in human cardiac fibroblasts. J Biol Chem 2008;283:8211-7.
88. Feng T, Meng J, Kou S, et al. CCN1-induced cellular senescence promotes heart regeneration. Circulation 2019;139:2495-8.
89. Aw D, Silva AB, Palmer DB. Immunosenescence: emerging challenges for an ageing population. Immunology 2007;120:435-46.
90. Childs BG, Baker DJ, Wijshake T, Conover CA, Campisi J, van Deursen JM. Senescent intimal foam cells are deleterious at all stages of atherosclerosis. Science 2016;354:472-7.
91. Yu HT, Park S, Shin EC, Lee WW. T cell senescence and cardiovascular diseases. Clin Exp Med 2016;16:257-63.
92. Franceschi C, Bonafè M, Valensin S, et al. Inflamm-aging: an evolutionary perspective on immunosenescence. Ann N Y Acad Sci 2000;908:244-54.
93. Varricchi G, Bencivenga L, Poto R, Pecoraro A, Shamji MH, Rengo G. The emerging role of T follicular helper (TFH) cells in aging: Influence on the immune frailty. Ageing Res Rev 2020;61:101071.
94. Ramos GC, van den Berg A, Nunes-Silva V, et al. Myocardial aging as a T-cell-mediated phenomenon. Proc Natl Acad Sci USA 2017;114:E2420-9.
95. Tae Yu H, Youn JC, Lee J, et al. Characterization of CD8+CD57+ T cells in patients with acute myocardial infarction. Cell Mol Immunol 2015;12:466-73.
96. Youn JC, Yu HT, Lim BJ, et al. Immunosenescent CD8+ T cells and C-X-C chemokine receptor type 3 chemokines are increased in human hypertension. Hypertension 2013;62:126-33.
97. Lagnado A, Leslie J, Ruchaud-Sparagano MH, et al. Neutrophils induce paracrine telomere dysfunction and senescence in ROS-dependent manner. EMBO J 2021;40:e106048.
98. Donato AJ, Morgan RG, Walker AE, Lesniewski LA. Cellular and molecular biology of aging endothelial cells. J Mol Cell Cardiol 2015;89:122-35.
99. Weber C, Noels H. Atherosclerosis: current pathogenesis and therapeutic options. Nat Med 2011;17:1410-22.
100. Abbas M, Jesel L, Auger C, et al. Endothelial microparticles from acute coronary syndrome patients induce premature coronary artery endothelial cell aging and thrombogenicity: role of the ang II/AT1 receptor/NADPH oxidase-mediated activation of MAPKs and PI3-kinase pathways. Circulation 2017;135:280-96.
101. Rossman MJ, Kaplon RE, Hill SD, et al. Endothelial cell senescence with aging in healthy humans: prevention by habitual exercise and relation to vascular endothelial function. Am J Physiol Heart Circ Physiol 2017;313:H890-5.
102. Grootaert MOJ, Moulis M, Roth L, et al. Vascular smooth muscle cell death, autophagy and senescence in atherosclerosis. Cardiovasc Res 2018;114:622-34.
103. Matthews C, Gorenne I, Scott S, et al. Vascular smooth muscle cells undergo telomere-based senescence in human atherosclerosis: effects of telomerase and oxidative stress. Circ Res 2006;99:156-64.
104. Wang J, Uryga AK, Reinhold J, et al. Vascular smooth muscle cell senescence promotes atherosclerosis and features of plaque vulnerability. Circulation 2015;132:1909-19.
105. Gardner SE, Humphry M, Bennett MR, Clarke MCH. Senescent vascular smooth muscle cells drive inflammation through an interleukin-1α-dependent senescence-associated secretory phenotype. Arterioscler Thromb Vasc Biol 2015;35:1963-74.
106. Kunieda T, Minamino T, Nishi JI, et al. Angiotensin II induces premature senescence of vascular smooth muscle cells and accelerates the development of atherosclerosis via a p21-dependent pathway. Circulation 2006;114:953-60.
107. Cahu J, Bustany S, Sola B. Senescence-associated secretory phenotype favors the emergence of cancer stem-like cells. Cell Death Dis 2012;3:e446.
108. Muhandiramge J, Zalcberg JR, van Londen GJ, et al. Cardiovascular disease in adult cancer survivors: a review of current evidence, strategies for prevention and management, and future directions for cardio-oncology. Curr Oncol Rep 2022;24:1579-92.
109. Lipshultz SE, Adams MJ, Colan SD, et al. Long-term cardiovascular toxicity in children, adolescents, and young adults who receive cancer therapy: pathophysiology, course, monitoring, management, prevention, and research directions: a scientific statement from the American Heart Association. Circulation 2013;128:1927-95.
110. Lipshultz SE, Lipsitz SR, Mone SM, et al. Female sex and higher drug dose as risk factors for late cardiotoxic effects of doxorubicin therapy for childhood cancer. N Engl J Med 1995;332:1738-43.
111. Altieri P, Barisione C, Lazzarini E, et al. Testosterone antagonizes doxorubicin-induced senescence of cardiomyocytes. J Am Heart Assoc 2016;5:e002383.
112. Adão R, de Keulenaer G, Leite-Moreira A, Brás-Silva C. Cardiotoxicity associated with cancer therapy: pathophysiology and prevention [Cardiotoxicidade associada à terapêutica oncológica: mecanismos fisiopatológicos e estratégias de prevenção]. Revista Portuguesa Cardiologia 2013;32:395-409.
113. Jha S, Sharma PK, Malviya R. Hyperthermia: role and risk factor for cancer treatment. Achiev Life Sci 2016;10:161-7.
114. Gent DG, Saif M, Dobson R, Wright DJ. Cardiovascular disease after hematopoietic stem cell transplantation in adults: JACC: CardioOncology state-of-the-art review. JACC CardioOncol 2024;6:475-95.
115. Miller KD, Siegel RL, Lin CC, et al. Cancer treatment and survivorship statistics, 2016. CA Cancer J Clin 2016;66:271-89.
116. Ramadan R, Baatout S, Aerts A, Leybaert L. The role of connexin proteins and their channels in radiation-induced atherosclerosis. Cell Mol Life Sci 2021;78:3087-103.
117. Belzile-Dugas E, Eisenberg MJ. Radiation-induced cardiovascular disease: review of an underrecognized pathology. J Am Heart Assoc 2021;10:e021686.
118. Shimizu Y, Kodama K, Nishi N, et al. Radiation exposure and circulatory disease risk: Hiroshima and Nagasaki atomic bomb survivor data, 1950-2003. BMJ 2010;340:b5349.
119. Yamada M, Wong FL, Fujiwara S, Akahoshi M, Suzuki G. Noncancer disease incidence in atomic bomb survivors, 1958-1998. Radiat Res 2004;161:622-32.
120. Little MP, Azizova TV, Richardson DB, et al. Ionising radiation and cardiovascular disease: systematic review and meta-analysis. BMJ 2023;380:e072924.
121. Tapio S. Pathology and biology of radiation-induced cardiac disease. J Radiat Res 2016;57:439-48.
122. Sallam M, Benotmane MA, Baatout S, Guns PJ, Aerts A. Radiation-induced cardiovascular disease: an overlooked role for DNA methylation? Epigenetics 2022;17:59-80.
123. Brosius III FC, Waller BF, Roberts WC. Radiation heart disease. Analysis of 16 young (aged 15 to 33 years) necropsy patients who received over 3,500 rads to the heart. Am J Med 1981;70:519-30.
124. Zhang B, Wang Y, Pang X, Su Y, Ai G, Wang T. ER stress induced by ionising radiation in IEC-6 cells. Int J Radiat Biol 2010;86:429-35.
125. Wang Y, Boerma M, Zhou D. Ionizing radiation-induced endothelial cell senescence and cardiovascular diseases. Radiat Res 2016;186:153-61.
126. Yakes FM, Van Houten B. Mitochondrial DNA damage is more extensive and persists longer than nuclear DNA damage in human cells following oxidative stress. Proc Natl Acad Sci USA 1997;94:514-9.
127. Wang KX, Ye C, Yang X, Ma P, Yan C, Luo L. New insights into the understanding of mechanisms of radiation-induced heart disease. Curr Treat Options Oncol 2023;24:12-29.
128. Schmitt K, Tulzer G, Merl M, et al. Early detection of doxorubicin and daunorubicin cardiotoxicity by echocardiography: diastolic versus systolic parameters. Eur J Pediatr 1995;154:201-4.
129. Ryberg M, Nielsen D, Skovsgaard T, Hansen J, Jensen BV, Dombernowsky P. Epirubicin cardiotoxicity: an analysis of 469 patients with metastatic breast cancer. J Clin Oncol 1998;16:3502-8.
130. Anderlini P, Benjamin RS, Wong FC, et al. Idarubicin cardiotoxicity: a retrospective study in acute myeloid leukemia and myelodysplasia. J Clin Oncol 1995;13:2827-34.
131. Swain SM, Whaley FS, Ewer MS. Congestive heart failure in patients treated with doxorubicin: a retrospective analysis of three trials. Cancer 2003;97:2869-79.
132. Cappetta D, Rossi F, Piegari E, et al. Doxorubicin targets multiple players: a new view of an old problem. Pharmacol Res 2018;127:4-14.
133. Muller C, Chatelut E, Gualano V, et al. Cellular pharmacokinetics of doxorubicin in patients with chronic lymphocytic leukemia: comparison of bolus administration and continuous infusion. Cancer Chemother Pharmacol 1993;32:379-84.
134. Linders AN, Dias IB, López Fernández T, Tocchetti CG, Bomer N, Van der Meer P. A review of the pathophysiological mechanisms of doxorubicin-induced cardiotoxicity and aging. NPJ Aging 2024;10:9.
135. Sturgeon K, Schadler K, Muthukumaran G, et al. Concomitant low-dose doxorubicin treatment and exercise. Am J Physiol Regul Integr Comp Physiol 2014;307:R685-92.
136. Choi WG, Kim DK, Shin Y, et al. Liquid chromatography-tandem mass spectrometry for the simultaneous determination of doxorubicin and its metabolites doxorubicinol, doxorubicinone, doxorubicinolone, and 7-deoxydoxorubicinone in mouse plasma. Molecules 2020;25:1254.
137. Huang R, Zhou PK. DNA damage repair: historical perspectives, mechanistic pathways and clinical translation for targeted cancer therapy. Signal Transduct Target Ther 2021;6:254.
138. Abdelgawad IY, Sadak KT, Lone DW, Dabour MS, Niedernhofer LJ, Zordoky BN. Molecular mechanisms and cardiovascular implications of cancer therapy-induced senescence. Pharmacol Ther 2021;221:107751.
139. Bielak-Zmijewska A, Wnuk M, Przybylska D, et al. A comparison of replicative senescence and doxorubicin-induced premature senescence of vascular smooth muscle cells isolated from human aorta. Biogerontology 2014;15:47-64.
140. d'Adda di Fagagna F. Living on a break: cellular senescence as a DNA-damage response. Nat Rev Cancer 2008;8:512-22.
141. Hodjat M, Haller H, Dumler I, Kiyan Y. Urokinase receptor mediates doxorubicin-induced vascular smooth muscle cell senescence via proteasomal degradation of TRF2. J Vasc Res 2013;50:109-23.
142. Linders AN, Dias IB, Ovchinnikova ES, et al. Evaluation of senescence and its prevention in doxorubicin-induced cardiotoxicity using dynamic engineered heart tissues. JACC CardioOncol 2023;5:298-315.
143. Maejima Y, Adachi S, Ito H, Hirao K, Isobe M. Induction of premature senescence in cardiomyocytes by doxorubicin as a novel mechanism of myocardial damage. Aging Cell 2008;7:125-36.
144. Ruan Y, Dong C, Patel J, et al. SIRT1 suppresses doxorubicin-induced cardiotoxicity by regulating the oxidative stress and p38MAPK pathways. Cell Physiol Biochem 2015;35:1116-24.
146. Agudelo D, Bourassa P, Bérubé G, Tajmir-Riahi HA. Intercalation of antitumor drug doxorubicin and its analogue by DNA duplex: structural features and biological implications. Int J Biol Macromol 2014;66:144-50.
147. Chen NT, Wu CY, Chung CY, et al. Probing the dynamics of doxorubicin-DNA intercalation during the initial activation of apoptosis by fluorescence lifetime imaging microscopy (FLIM). PLoS One 2012;7:e44947.
148. Skladanowski A, Konopa J. Interstrand DNA crosslinking induced by anthracyclines in tumour cells. Biochem Pharmacol 1994;47:2269-78.
149. Zhang S, Liu X, Bawa-Khalfe T, et al. Identification of the molecular basis of doxorubicin-induced cardiotoxicity. Nat Med 2012;18:1639-42.
150. Thornton TM, Rincon M. Non-classical p38 map kinase functions: cell cycle checkpoints and survival. Int J Biol Sci 2009;5:44-52.
151. Khan SY, Awad EM, Oszwald A, et al. Premature senescence of endothelial cells upon chronic exposure to TNFα can be prevented by N-acetyl cysteine and plumericin. Sci Rep 2017;7:39501.
152. Carvalho FS, Burgeiro A, Garcia R, Moreno AJ, Carvalho RA, Oliveira PJ. Doxorubicin-induced cardiotoxicity: from bioenergetic failure and cell death to cardiomyopathy. Med Res Rev 2014;34:106-35.
153. Peoples JN, Saraf A, Ghazal N, Pham TT, Kwong JQ. Mitochondrial dysfunction and oxidative stress in heart disease. Exp Mol Med 2019;51:1-13.
154. Sangomla S, Saifi MA, Khurana A, Godugu C. Nanoceria ameliorates doxorubicin induced cardiotoxicity: possible mitigation via reduction of oxidative stress and inflammation. J Trace Elem Med Biol 2018;47:53-62.
156. Ferreira A, Cunha-Oliveira T, Simões RF, et al. Altered mitochondrial epigenetics associated with subchronic doxorubicin cardiotoxicity. Toxicology 2017;390:63-73.
157. Lesnefsky EJ, Chen Q, Hoppel CL. Mitochondrial metabolism in aging heart. Circ Res 2016;118:1593-611.
158. Pillai VB, Kanwal A, Fang YH, et al. Honokiol, an activator of Sirtuin-3 (SIRT3) preserves mitochondria and protects the heart from doxorubicin-induced cardiomyopathy in mice. Oncotarget 2017;8:34082-98.
159. De Falco E, Carnevale R, Pagano F, et al. Role of NOX2 in mediating doxorubicin-induced senescence in human endothelial progenitor cells. Mech Ageing Dev 2016;159:37-43.
160. Ong G, Logue SE. Unfolding the interactions between endoplasmic reticulum stress and oxidative stress. Antioxidants 2023;12:981.
161. Guo RM, Xu WM, Lin JC, et al. Activation of the p38 MAPK/NF-κB pathway contributes to doxorubicin-induced inflammation and cytotoxicity in H9c2 cardiac cells. Mol Med Rep 2013;8:603-8.
162. Notarbartolo M, Poma P, Perri D, Dusonchet L, Cervello M, D’Alessandro N. Antitumor effects of curcumin, alone or in combination with cisplatin or doxorubicin, on human hepatic cancer cells. Analysis of their possible relationship to changes in NF-κB activation levels and in IAP gene expression. Cancer Lett 2005;224:53-65.
163. Fallah M, Mohammadi H, Shaki F, et al. Doxorubicin and liposomal doxorubicin induce senescence by enhancing nuclear factor kappa B and mitochondrial membrane potential. Life Sci 2019;232:116677.
164. Tilstra JS, Robinson AR, Wang J, et al. NF-κB inhibition delays DNA damage-induced senescence and aging in mice. J Clin Invest 2012;122:2601-12.
165. Mancilla TR, Davis LR, Aune GJ. Doxorubicin-induced p53 interferes with mitophagy in cardiac fibroblasts. PLoS One 2020;15:e0238856.
166. Bent EH, Gilbert LA, Hemann MT. A senescence secretory switch mediated by PI3K/AKT/mTOR activation controls chemoprotective endothelial secretory responses. Genes Dev 2016;30:1811-21.
167. Smogorzewska A, de Lange T. Different telomere damage signaling pathways in human and mouse cells. EMBO J 2002;21:4338-48.
168. Beauséjour CM, Krtolica A, Galimi F, et al. Reversal of human cellular senescence: roles of the p53 and p16 pathways. EMBO J 2003;22:4212-22.
169. Jacobs JJL, de Lange T. Significant role for p16INK4a in p53-independent telomere-directed senescence. Curr Biol 2004;14:2302-8.
170. Spallarossa P, Altieri P, Aloi C, et al. Doxorubicin induces senescence or apoptosis in rat neonatal cardiomyocytes by regulating the expression levels of the telomere binding factors 1 and 2. Am J Physiol Heart Circ Physiol 2009;297:H2169-81.
171. Roos CM, Zhang B, Palmer AK, et al. Chronic senolytic treatment alleviates established vasomotor dysfunction in aged or atherosclerotic mice. Aging Cell 2016;15:973-7.
172. Crouch J, Shvedova M, Thanapaul RJRS, Botchkarev V, Roh D. Epigenetic regulation of cellular senescence. Cells 2022;11:672.
173. Jones MJ, Goodman SJ, Kobor MS. DNA methylation and healthy human aging. Aging Cell 2015;14:924-32.
174. Ferreira LL, Cunha-Oliveira T, Veloso CD, Costa CF, Wallace KB, Oliveira PJ. Single nanomolar doxorubicin exposure triggers compensatory mitochondrial responses in H9c2 cardiomyoblasts. Food Chem Toxicol 2019;124:450-61.
175. Cruickshanks HA, McBryan T, Nelson DM, et al. Senescent cells harbour features of the cancer epigenome. Nat Cell Biol 2013;15:1495-506.
176. Song R, Yang Y, Lei H, et al. HDAC6 inhibition protects cardiomyocytes against doxorubicin-induced acute damage by improving α-tubulin acetylation. J Mol Cell Cardiol 2018;124:58-69.
177. Piotrowska I, Isalan M, Mielcarek M. Early transcriptional alteration of histone deacetylases in a murine model of doxorubicin-induced cardiomyopathy. PLoS One 2017;12:e0180571.
178. McIntyre RL, Daniels EG, Molenaars M, Houtkooper RH, Janssens GE. From molecular promise to preclinical results: HDAC inhibitors in the race for healthy aging drugs. EMBO Mol Med 2019;11:e9854.
179. Chaudhari U, Ellis JK, Wagh V, et al. Metabolite signatures of doxorubicin induced toxicity in human induced pluripotent stem cell-derived cardiomyocytes. Amino Acids 2017;49:1955-63.
180. Garcia MM, Guéant-Rodriguez RM, Pooya S, et al. Methyl donor deficiency induces cardiomyopathy through altered methylation/acetylation of PGC-1α by PRMT1 and SIRT1. J Pathol 2011;225:324-35.
181. Xiong S, Salazar G, Patrushev N, et al. Peroxisome proliferator-activated receptor γ coactivator-1α is a central negative regulator of vascular senescence. Arterioscler Thromb Vasc Biol 2013;33:988-98.
182. Li W, Cao J, Wang X, et al. Ferruginol restores SIRT1-PGC-1α-mediated mitochondrial biogenesis and fatty acid oxidation for the treatment of DOX-induced cardiotoxicity. Front Pharmacol 2021;12:773834.
183. Lamore SD, Kohnken RA, Peters MF, Kolaja KL. Cardiovascular toxicity induced by kinase inhibitors: mechanisms and preclinical approaches. Chem Res Toxicol 2020;33:125-36.
184. Wang H, Wang Y, Li J, et al. Three tyrosine kinase inhibitors cause cardiotoxicity by inducing endoplasmic reticulum stress and inflammation in cardiomyocytes. BMC Med 2023;21:147.
185. Tanriverdi O, Ates S, Sandal KK, Uylas S, Bosna IC, Alkan A. Left ventricular dysfunction associated with axitinib and nivolumab experience in an advanced renal cell carcinoma. J Oncol Pharm Pract 2020;26:1765-8.
186. Mongiardi MP, Radice G, Piras M, et al. Axitinib exposure triggers endothelial cells senescence through ROS accumulation and ATM activation. Oncogene 2019;38:5413-24.
187. Neves KB, Alves-Lopes R, Montezano AC, Touyz RM. Role of PARP and TRPM2 in VEGF inhibitor-induced vascular dysfunction. J Am Heart Assoc 2023;12:e027769.
188. Gu Y, Avolio E, Alvino VV, et al. The tyrosine kinase inhibitor Dasatinib reduces cardiac steatosis and fibrosis in obese, type 2 diabetic mice. Cardiovasc Diabetol 2023;22:214.
189. Singh AP, Umbarkar P, Tousif S, Lal H. Cardiotoxicity of the BCR-ABL1 tyrosine kinase inhibitors: emphasis on ponatinib. Int J Cardiol 2020;316:214-21.
190. Madonna R, Barachini S, Moscato S, et al. Sodium-glucose cotransporter type 2 inhibitors prevent ponatinib-induced endothelial senescence and disfunction: a potential rescue strategy. Vascul Pharmacol 2022;142:106949.
191. Madonna R, Pieragostino D, Cufaro MC, et al. Ponatinib induces vascular toxicity through the Notch-1 signaling pathway. J Clin Med 2020;9:820.
192. Madonna R, Pieragostino D, Cufaro MC, et al. Sex-related differential susceptibility to ponatinib cardiotoxicity and differential modulation of the Notch1 signalling pathway in a murine model. J Cell Mol Med 2022;26:1380-91.
193. Wang D, Xiao F, Feng Z, et al. Sunitinib facilitates metastatic breast cancer spreading by inducing endothelial cell senescence. Breast Cancer Res 2020;22:103.
194. Zhu Y, Xu L, Zhang J, et al. Sunitinib induces cellular senescence via p53/Dec1 activation in renal cell carcinoma cells. Cancer Sci 2013;104:1052-61.
195. Chu TF, Rupnick MA, Kerkela R, et al. Cardiotoxicity associated with tyrosine kinase inhibitor sunitinib. Lancet 2007;370:2011-9.
196. Hotta K, Tabata M, Kiura K, et al. Gefitinib induces premature senescence in non-small cell lung cancer cells with or without EGFR gene mutation. Oncol Rep 2007;17:313-7.
197. Gasek NS, Kuchel GA, Kirkland JL, Xu M. Strategies for targeting senescent cells in human disease. Nat Aging 2021;1:870-9.
198. Owens WA, Walaszczyk A, Spyridopoulos I, Dookun E, Richardson GD. Senescence and senolytics in cardiovascular disease: promise and potential pitfalls. Mech Ageing Dev 2021;198:111540.
199. Zhu Y, Tchkonia T, Fuhrmann-Stroissnigg H, et al. Identification of a novel senolytic agent, navitoclax, targeting the Bcl-2 family of anti-apoptotic factors. Aging Cell 2016;15:428-35.
200. Leverson JD, Phillips DC, Mitten MJ, et al. Exploiting selective BCL-2 family inhibitors to dissect cell survival dependencies and define improved strategies for cancer therapy. Sci Transl Med 2015;7:279ra40.
201. Chang J, Wang Y, Shao L, et al. Clearance of senescent cells by ABT263 rejuvenates aged hematopoietic stem cells in mice. Nat Med 2016;22:78-83.
202. Wilson WH, O'Connor OA, Czuczman MS, et al. Navitoclax, a targeted high-affinity inhibitor of BCL-2, in lymphoid malignancies: a phase 1 dose-escalation study of safety, pharmacokinetics, pharmacodynamics, and antitumour activity. Lancet Oncol 2010;11:1149-59.
203. AbbVie. A phase 2 open-label study evaluating tolerability and efficacy of navitoclax alone or in combination with Ruxolitinib in subjects with myelofibrosis (REFINE). 2017. Available from: https://clinicaltrials.gov/study/NCT03222609 [Last accessed on 14 Nov 2024].
204. Lérida-Viso A, Estepa-Fernández A, Morellá-Aucejo Á, et al. Pharmacological senolysis reduces doxorubicin-induced cardiotoxicity and improves cardiac function in mice. Pharmacol Res 2022;183:106356.
205. Zhu Y, Doornebal EJ, Pirtskhalava T, et al. New agents that target senescent cells: the flavone, fisetin, and the BCL-XL inhibitors, A1331852 and A1155463. Aging 2017;9:955-63.
206. Zhu Y, Tchkonia T, Pirtskhalava T, et al. The Achilles’ heel of senescent cells: from transcriptome to senolytic drugs. Aging Cell 2015;14:644-58.
207. Gonzales MM, Garbarino VR, Kautz TF, et al. Senolytic therapy in mild Alzheimer's disease: a phase 1 feasibility trial. Nat Med 2023;29:2481-8.
208. Wake Forest University Health Sciences. Phase II clinical trial to evaluate the safety and feasibility of senolytic therapy in Alzheimer’s disease. 2020. Available from: https://clinicaltrials.gov/study/NCT04685590 [Last accessed on 14 Nov 2024].
209. Yousefzadeh MJ, Zhu Y, McGowan SJ, et al. Fisetin is a senotherapeutic that extends health and lifespan. EBioMedicine 2018;36:18-28.
210. Gupta SC, Tyagi AK, Deshmukh-Taskar P, Hinojosa M, Prasad S, Aggarwal BB. Downregulation of tumor necrosis factor and other proinflammatory biomarkers by polyphenols. Arch Biochem Biophys 2014;559:91-9.
211. Huard CA, Gao X, Dey Hazra ME, et al. Effects of fisetin treatment on cellular senescence of various tissues and organs of old sheep. Antioxidants 2023;12:1646.
212. Jonsson Comprehensive Cancer Center. A phase II randomized placebo-controlled study of fisetin and exercise to prevent frailty in breast cancer survivors. 2023. Available from: https://clinicaltrials.gov/study/NCT06113016 [Last accessed on 14 Nov 2024].
213. Jonsson Comprehensive Cancer Center. A phase II randomized double-blind placebo-controlled study of fisetin to improve physical function in breast cancer survivors. 2022. Available from: https://clinicaltrials.gov/study/NCT05595499 [Last accessed on 14 Nov 2024].
214. Stojanović SD, Fiedler J, Bauersachs J, Thum T, Sedding DG. Senescence-induced inflammation: an important player and key therapeutic target in atherosclerosis. Eur Heart J 2020;41:2983-96.
215. Wang R, Yu Z, Sunchu B, et al. Rapamycin inhibits the secretory phenotype of senescent cells by a Nrf2-independent mechanism. Aging Cell 2017;16:564-74.
216. Singh M, Jensen MD, Lerman A, et al. Effect of low-dose rapamycin on senescence markers and physical functioning in older adults with coronary artery disease: results of a pilot study. J Frailty Aging 2016;5:204-7.
217. Kulkarni AS, Gubbi S, Barzilai N. Benefits of metformin in attenuating the hallmarks of aging. Cell Metab 2020;32:15-30.
218. Albert Einstein College of Medicine. Metformin in Longevity Study (MILES). 2015. Available from: https://clinicaltrials.gov/study/NCT02432287 [Last accessed on 14 Nov 2024].
219. University of Utah. Metformin to prevent inactivity-induced loss of muscle health during aging. 2017. Available from: https://clinicaltrials.gov/study/NCT03107884 [Last accessed on 14 Nov 2024].
220. University of New Mexico. A double-blind, placebo-controlled trial of anti-aging, pro-autophagy effects of metformin in adults with prediabetes. 2017. Available from: https://clinicaltrials.gov/study/NCT03309007 [Last accessed on 14 Nov 2024].
221. Kulkarni AS, Brutsaert EF, Anghel V, et al. Metformin regulates metabolic and nonmetabolic pathways in skeletal muscle and subcutaneous adipose tissues of older adults. Aging Cell 2018;17:e12723.
222. Alimbetov D, Davis T, Brook AJ, et al. Suppression of the senescence-associated secretory phenotype (SASP) in human fibroblasts using small molecule inhibitors of p38 MAP kinase and MK2. Biogerontology 2016;17:305-15.
223. Zhang L, Zhao J, Mu X, et al. Novel small molecule inhibition of IKK/NF-κB activation reduces markers of senescence and improves healthspan in mouse models of aging. Aging Cell 2021;20:e13486.
224. Yu H, Pardoll D, Jove R. STATs in cancer inflammation and immunity: a leading role for STAT3. Nat Rev Cancer 2009;9:798-809.
225. Xu M, Tchkonia T, Ding H, et al. JAK inhibition alleviates the cellular senescence-associated secretory phenotype and frailty in old age. Proc Natl Acad Sci USA 2015;112:E6301-10.
226. Laberge RM, Zhou L, Sarantos MR, et al. Glucocorticoids suppress selected components of the senescence-associated secretory phenotype. Aging Cell 2012;11:569-78.
227. Varghese LN, Schwenke DO, Katare R. Role of noncoding RNAs in cardiac ageing. Front Cardiovasc Med 2023;10:1142575.
228. Devaux Y, Zangrando J, Schroen B, et al. Long noncoding RNAs in cardiac development and ageing. Nat Rev Cardiol 2015;12:415-25.
229. Suski JM, Karkucinska-Wieckowska A, Lebiedzinska M, et al. p66Shc aging protein in control of fibroblasts cell fate. Int J Mol Sci 2011;12:5373-89.
230. Migliaccio E, Giorgio M, Mele S, et al. The p66shc adaptor protein controls oxidative stress response and life span in mammals. Nature 1999;402:309-13.
231. Napoli C, Martin-Padura I, de Nigris F, et al. Deletion of the p66Shc longevity gene reduces systemic and tissue oxidative stress, vascular cell apoptosis, and early atherogenesis in mice fed a high-fat diet. Proc Natl Acad Sci USA 2003;100:2112-6.
232. Rezk NA, Lashin MB, Sabbah NA. MiRNA 34-a regulate SIRT-1 and Foxo-1 expression in endometriosis. Noncoding RNA Res 2021;6:35-41.
233. Fomison-Nurse I, Saw EEL, Gandhi S, et al. Diabetes induces the activation of pro-ageing miR-34a in the heart, but has differential effects on cardiomyocytes and cardiac progenitor cells. Cell Death Differ 2018;25:1336-49.
234. Wang H, Bei Y, Shi J, Xiao J, Kong X. Non-coding RNAs in cardiac aging. Cell Physiol Biochem 2015;36:1679-87.
235. Du WW, Li X, Li T, et al. The microRNA miR-17-3p inhibits mouse cardiac fibroblast senescence by targeting Par4. J Cell Sci 2015;128:293-304.
236. Jazbutyte V, Fiedler J, Kneitz S, et al. MicroRNA-22 increases senescence and activates cardiac fibroblasts in the aging heart. Age 2013;35:747-62.
237. Deckx S, Heggermont W, Carai P, et al. Osteoglycin prevents the development of age-related diastolic dysfunction during pressure overload by reducing cardiac fibrosis and inflammation. Matrix Biol 2018;66:110-24.
238. Li H, Zhang P, Li F, et al. Plasma miR-22-5p, miR-132-5p, and miR-150-3p are associated with acute myocardial infarction. Biomed Res Int 2019;2019:5012648.
239. Weigl M, Krammer TL, Pultar M, et al. Profiling microRNA expression during senescence and aging: mining for a diagnostic tool of senescent-cell burden. bioRxiv 2024.
240. Tripathi V, Shen Z, Chakraborty A, et al. Long noncoding RNA MALAT1 controls cell cycle progression by regulating the expression of oncogenic transcription factor B-MYB. PLoS Genet 2013;9:e1003368.
241. Ratti M, Lampis A, Ghidini M, et al. MicroRNAs (miRNAs) and long non-coding RNAs (lncRNAs) as new tools for cancer therapy: first steps from bench to bedside. Target Oncol 2020;15:261-78.
242. Fang Y, Xu Y, Wang R, et al. Recent advances on the roles of LncRNAs in cardiovascular disease. J Cell Mol Med 2020;24:12246-57.
243. Suda M, Shimizu I, Katsuumi G, et al. Senolytic vaccination improves normal and pathological age-related phenotypes and increases lifespan in progeroid mice. Nat Aging 2021;1:1117-26.
244. Johmura Y, Yamanaka T, Omori S, et al. Senolysis by glutaminolysis inhibition ameliorates various age-associated disorders. Science 2021;371:265-70.
245. Cheng JW, Rybak I. Use of digoxin for heart failure and atrial fibrillation in elderly patients. Am J Geriatr Pharmacother 2010;8:419-27.
246. Triana-Martínez F, Picallos-Rabina P, Da Silva-Álvarez S, et al. Identification and characterization of Cardiac Glycosides as senolytic compounds. Nat Commun 2019;10:4731.
Cite This Article

How to Cite
Alshoubaki, Y. K.; Grüterich V.; Zollet, V.; Kuster, G. M. The role of senescence in cancer therapy-associated cardiovascular toxicity. J. Cardiovasc. Aging. 2024, 4, 23. http://dx.doi.org/10.20517/jca.2024.14
Download Citation
Export Citation File:
Type of Import
Tips on Downloading Citation
Citation Manager File Format
Type of Import
Direct Import: When the Direct Import option is selected (the default state), a dialogue box will give you the option to Save or Open the downloaded citation data. Choosing Open will either launch your citation manager or give you a choice of applications with which to use the metadata. The Save option saves the file locally for later use.
Indirect Import: When the Indirect Import option is selected, the metadata is displayed and may be copied and pasted as needed.
About This Article
Copyright
Data & Comments
Data
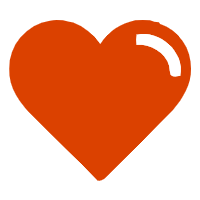
Comments
Comments must be written in English. Spam, offensive content, impersonation, and private information will not be permitted. If any comment is reported and identified as inappropriate content by OAE staff, the comment will be removed without notice. If you have any queries or need any help, please contact us at support@oaepublish.com.