Use of a fluorescent molecular rotor probe for nanoplastics assessment in epiphytic biofilms growing on submerged vegetation of Lake Saint Pierre (St. Lawrence River)
Abstract
Studies on plastic pollution conducted in freshwaters mainly focused on monitoring plastic debris in the water column or in the sediments. Few studies have investigated the occurrence of plastic debris in benthic biofilms (periphyton). Yet, algal biofilms may potentially act as a sink for plastic debris, trapping it within their mucilaginous or filamentous matrix. Biofilms may also represent a source of plastic debris by sloughing when they become senescent. In addition, plastic debris accumulated within biofilms may enter the food web via primary consumers. Considering these observations, this study aims to quantify nanoplastics (NPs) accumulated in biofilms growing on aquatic vegetation from Lake Saint Pierre (LSP), a fluvial lake of the St. Lawrence River, and its archipelago. Biofilms were removed from submerged plants and the presence of NPs was assessed by spectrophotometry using the fluorescent molecular rotor probe 9(dicyanovinyl)-julolidine (DCVJ). The results of this study confirm the biofilms’ ability to act as a sink for NPs. Despite the fact that the determination of the absolute nanoparticle number and size distribution remains a challenge, we estimated a median concentration of 1.05 × 109 NP/mg of biofilm dry weight (DW) when using 100 nm polystyrene beads for calibration. Concentrations were significantly different between water masses, with higher concentrations in samples collected in the two lateral water masses compared to the central water mass. Our study provides, for the first time, a quantitative assessment of NPs from epiphytic biofilms in a large river under the influence of anthropogenic sources.
Keywords
INTRODUCTION
Since the year 1950, when global plastic production was around 0.5 million tons[1], its annual production has grown steadily, reaching 400 million tons per year in 2022[2]. North America produced 17% of these plastics in 2022[2]. With increasing production, extensive usage of plastic products, as well as poor waste management combined with insufficient regulation, plastic pollution raised global concerns as debris [i.e., mesoplastics 5 to 25 mm, microplastics (MPs) 1 µm to 5 mm, and nanoplastics (NPs) ≤ 1 µm[3]] has been detected in all ecosystems worldwide[4]. For instance, hundreds of research articles assessed the presence and fate of plastic debris in the aquatic environment over the past decade, with a particular focus on MPs in marine ecosystems and in the water column[5]. In the water compartment, plastic debris of 0.45 to 505 µm in size has been detected in concentrations ranging between 3 × 10-8 to 2 particles per mL[6]. Several studies focusing on marine ecosystems highlighted the adverse effects of plastic debris on the biota. Plastic pollution can cause physical damage, such as the entanglement of animal appendages or the obstruction of their digestive tract[7]. Biological and chemical effects such as oxidative stress or DNA damage have also been reported[6], and have been related to the presence of additives compounds such as plasticizers used during plastic production or to the presence of sorbed contaminants[8].
Compared to the marine environment, there are fewer studies that investigated the occurrence of plastic debris in freshwater ecosystems and their ecotoxicity[6]. However, streams and rivers are the major pathways for plastic debris into marine ecosystems[9], and studies suggest that these freshwaters may be at least as contaminated as marine waters[10]. For example, the sediment compartment of the St. Lawrence River is among the 25% most polluted in the world, with concentrations ranging from 65 to 7,562 plastic particles per kg dry weight (DW)[11]. Concentrations ranging from 48 to 187 particles per L were recorded in the water column of the Amsterdam canal (the Netherlands), which was higher than concentrations recorded in the North Sea coast (the Netherlands), where the mean concentration recorded was 27 particles per L[12].
Studies conducted in freshwaters mainly focused on monitoring plastic debris concentrations in the water column or in the sediments, while their occurrence and abundance in periphytic biofilms (or periphyton) have been largely overlooked. Periphyton is a key compartment at the base of aquatic and terrestrial food webs; it is a consortium of viruses, archaea, bacteria, algae, protozoa, fungi, and meiofauna, embedded in a matrix of extracellular polymeric substances. Periphyton colonizes all types of substrates exposed to light[13]. This biological matrix may function as a sink for plastic debris as particles get trapped and incorporated and may also represent a source of plastic particles for higher trophic levels[14]. Trapped plastic debris may have several ecotoxicological consequences on the microorganisms composing the periphyton, as reviewed in Guasch et al. (2022)[15]. For example, a study by Merbt et al. (2022) conducted on periphyton exposed to virgin and aged polyethylene (PE) beads (1-4 µm, 0.96 g/mL) for 28 days revealed their incorporation in the periphyton and showed a significant shift in microbial community composition[16]. A study by Miao et al. (2019) observed that periphyton exposed for 3 h to polystyrene beads (0.1 µm, 0.1 mg/mL) led to a significant decrease in chlorophyll concentration and impaired activity of functional enzymes, such as β-glucosidase and leucine aminopeptidase[17]. Plastic debris also acts as vectors for contaminants and may consequently influence their bioavailability[18] for the microorganisms of the periphyton and for consumers.
Biofilms influence the degradation of plastic particles by promoting their fragmentation by microorganisms that secrete enzymes capable of breaking covalent bonds[14]. Plastic debris accumulated within the periphyton may enter the food web via primary consumers feeding on this basal resource[19]. Considering that periphytic biofilms might act as a sink of plastic particles, dietary exposure of consumers might be more important than direct exposition through the water column[15]. Despite the key role periphytic biofilms play in freshwaters, little is known about their ability to trap and accumulate plastic particles[14]. To our knowledge, the article by Wang et al. (2023) is the only field study that provides evidence supporting the hypothesis that periphyton can incorporate plastic debris[20]. They recorded MPs, mainly fibers, in concentrations ranging from 419 to 1,314 items/m2 in the CaoE River, China[20]. The authors focused on particle sizes in the mm range (≤ 0.5, 0.5-1, 1-2, 2-5 mm) as plastic debris was handpicked under an optical stereomicroscope prior to identification through Raman microscope spectroscopy.
To this date, less research has focused on NPs as compared to MPs; nanosize particles require complex analytical methods to be accurately quantified[21] and only a few studies measured particles smaller than
Based on the scarcity of studies conducted to address plastic pollution in freshwaters, especially within the biofilm compartment, additional knowledge is needed to assess the extent to which this primary resource may act as a sink for NPs and as a source of contamination to consumers. The main objective of this study was to estimate NPs accumulated in periphyton growing on aquatic vegetation (i.e., epiphyton) from Lake Saint Pierre (LSP), a fluvial lake of the St. Lawrence River, and its archipelago (Quebec, Canada). LSP is an exceptionally rich ecosystem, both biologically and economically[27]. This widening of the St. Lawrence River is recognized as an ecosystem of international importance under the Ramsar Convention and is identified as a World Biosphere Reserve by UNESCO[28]. Plant beds of submerged/rooted aquatic vegetation are one of the key components of LSP, as they play an essential role in regulating various physical parameters such as current velocity and sedimentation of suspended particulate matter[29]. These dense plant communities provide essential habitats for the survival of many species of invertebrates and fish[29], and the epiphyton growing on this vegetation is a key component of the food web as several consumers rely on this resource[30]. Aquatic plants also form the basis of waterfowl diets[31], which may be exposed to NPs accumulated within the epiphyton.
Seventy percent of the water flowing through LSP comes from Lake Ontario[27]. This water is of relatively good quality and mainly flows through the navigation channel[32]. The shallower areas of the lake are thus isolated by this central water mass and show degraded water quality due to certain highly impacted tributaries entering the lake[33]. Because the main sources of water coming into LSP mix very little[34], they form three distinct water masses with different physico-chemical characteristics. The different origins of the three water masses and their water quality led to the hypothesis that NP concentrations accumulating in the epiphyton will differ depending on sampling locations within the LSP system (north, south, and central water masses). A second hypothesis was formulated around the upstream-downstream longitudinal gradient, where NP concentrations were projected to be higher in the epiphyton collected at the upstream stations compared to downstream stations. This hypothesis was based on the assumption that dense aquatic vegetation will favor NPs accumulation in the epiphyton and thus act as a filter for plastic particles.
The secondary objective of this study was to explore the feasibility of assessing polystyrene-like NPs (PSNPs) in the epiphyton by spectrophotometry using the fluorescent molecular rotor probe 9(dicyanovinyl)-julolidine (DCVJ). In this study, NPs detection was based on the probe’s sensitivity to changes in hydrophobicity[35,36]. Briefly, a hydrophobic interaction between the probe and polystyrene nanoplastic beads (PSN) occurs at the PSN’s surface, which produces a fluorescent signal[36]. After calibration with PSN, this signal is correlated to the presence of PSN and/or PSNP polymers, and its intensity is proportional to their concentration. The method allows for relative quantification of NPs in biofilm samples with PSN as the reference polymer. This method was previously used to assess PSN in diverse biological samples such as mussels[35-37] or radish sprout[35] and was tested here for the first time in the complex and heterogenous periphyton matrix.
MATERIALS AND METHODS
Study area
Lake Saint Pierre [Figure 1], stretching from Sorel to Trois-Rivières, is a widening of the St. Lawrence River and is characterized by a lentic hydrology (except for the central water mass)[38]. The system is 32 km long and
Figure 1. Distribution of water masses within Lake Saint-Pierre (Quebec, Canada) and the stations visited in 2023. Out of the 14 stations sampled, nine were located in the lake’s archipelago area (stations A1 to A9), three on the south shore (stations S1, S2, and S3), and two on the north shore (stations N1 and N2). Nine stations (A1 to A4, A6, A8, A9, N1, and N2) were located in the northern water body, three (A5, A7, and S1) in the central water body and two (S2 and S3) in the southern water body.
Biofilm sampling and water physicochemistry
In August 2023, 14 stations were visited across LSP and its archipelago. To minimize secondary contamination of samples with plastic debris, field staff were dressed in cotton clothing and used sampling tools made of metal or glass. No gloves were used during sampling to avoid potential contamination with plastic-like material. Biofilm samples were collected on submerged plants such as Vallisneria americana, Potamogeton pusillus, and Potamogeton perfoliatus. Submerged vegetation was harvested using a metal rake and transferred to a steel bucket containing 750 mL of lake water. Plants were then manually vigorously shaken to detach the epiphython. The content was then transferred into 1-liter glass jars after filtration on a 0.5 mm pore size metal sieve to remove plant debris and large invertebrates. Samples were preserved in coolers in the field and stored at 4 °C in the dark until arrival at the laboratory. In order to obtain representative samples, each of the 14 stations was sampled along a transect of three sampling points that were 10-20 meters apart. Conductivity, temperature, and pH were measured on site using the ORION Thermo Scientific multi-probe field instrument [Supplementary Table 1]. Once in the laboratory, biofilm samples were oven-dried at 50 °C to preserve the physicochemistry of potential plastic polymers[41], and stored at room temperature in obscurity until further analyses.
Sample digestion and detection of PSNPs by spectrophotometry
A subsample (referred to as samples hereafter) from each of the 42 biofilm samples (14 stations × 3 points per transect) was rehydrated in ultrapure water (5 mL per gram of dry mass) and manually stirred with a stainless steel spatula. To ensure complete rehydration, samples were placed on an orbital shaker at 300 rpm for one week (room temperature in obscurity). Afterwards, potassium hydroxide (KOH 20%) was added to each sample (v/v for a 10% final KOH concentration) to digest organic content[42] without degrading PSN, and the samples were left for agitation for one additional week after thorough vortexing. After one week under agitation, the digestates were left to stand for 3 h to allow for microparticles to settle. The supernatants were then collected for analysis. Potassium dihydrogen phosphate (KH2PO4, pH 6.5, 100 mM) was added (0.5 v/v) to neutralize KOH and avoid interference with the quantification method[42]. Levels of NPs were then estimated by spectrophotometry using the fluorescent molecular rotor probe DCVJ.
The commercial DCVJ probe (Sigma Aldrich, CAS 58293-56-4) was dissolved in methanol at a concentration of 1 mM to serve as a stock solution. The stock solution was diluted at 0.4 μM in ultrapure water for a daily solution used for fluorescence analysis. A volume of 30 µL collected from the sample’s supernatant was mixed with 160 μL of DCVJ probe and 10 μL of ultrapure water in 96-well microplates. The fluorescence intensity of the microplates was analyzed using a microplate reader (Agilent, BioTek, Synergy Neo 2) with the excitation wavelength at 450 nm and the emission wavelength at 620 nm. PSNPs detected in biofilm samples were assumed to have a maximum size of 100 nm as standard solutions of PSN beads of 50 and 100 nm (Polyscience, Polybead® Microspheres) were used for method calibration [Supplementary Figure 1]. Biofilm NP concentrations were calculated using the 100 nm low concentration calibration curve as they fit best the fluorescence intensity range, except for station S2 which had a higher fluorescence than the range provided by the 100nm low concentration curve, and as such levels were calculated with the
Quality assurance and quality control of the DCVJ probe assay
Several procedural controls were implemented in the protocol and were analyzed with the samples to assess (1) the potential contamination during the sample treatment process (i.e., negative controls); and (2) the efficiency of PSN recovery during the digestion process (digestion controls). Negative controls consisted of ultra-pure water samples that were digested using the same protocol as for biofilm samples, and the digestion controls were suspensions of PSN of a known theoretical concentration suspended in deionized water (8.41 × 1013 50 nm PSN/mL; 1.15 × 1013 100 nm PSN/mL) and digested also using the same protocol as for biofilm samples. Deionized water samples (water blanks) were analyzed to ensure the specificity of the DCVJ probe with PSN and to assess the background noise. Biological controls were performed during the method development and involved spiked biofilm samples (pooled biofilm samples, and the addition of a known theoretical concentration of 8.35 × 1012 PSN/mL) to assess fluorescence signal in the presence of the biofilm matrix and potential interactions. As for the limit of detection (LOD), its theoretical value (blank standard deviation × 2) was estimated at 65 ng/mL and the blank standard consisted of the DCVJ probe diluted in water (Gagné, 2019). Standard curves using suspensions of PSN (50 and 100 nm) were generated to validate the linearity of the signal using the DCVJ probe (R2 ranged between 0.92 and 0.99 depending on particle size; Supplementary Figure 1).
Data representation and analysis
Mapping of the sampling stations at the scale of LSP was carried out using QGIS-Server, QGIS software (version 3.26.1). Estimated levels of PSNPs in the biofilms growing on submerged vegetation at each station were graphically presented to allow for inter-station comparison, and a Pearson correlation was conducted to explore a potential upstream-downstream longitudinal gradient. Sampling stations were subsequently classified according to their respective water masses (i.e., northern, central, or southern water mass). Differences in terms of PSNP concentrations between the three water masses were assessed by a Kruskal-Wallis test followed by a Dunn post-hoc test using the FSA package. Significant differences were set at P < 0.05 and statistical analyses were conducted using the softwares R (version 4.3.0) and R studio (version 2024.04.0+735).
RESULTS
Quality assurance and quality control
Water blanks and negative controls used for the quality assurance and quality control (QAQC) assay did not emit any fluorescent signal (values not shown), contrary to samples containing PSN (i.e., digestion and biological controls). No autofluorescence from PSN controls was measured at 450:620 nm in the absence of DCVJ, indicating that the observed fluorescent signal in the presence of both PSN and DCVJ was specific to their interaction. Hence, biological controls emitted a fluorescent signal that was not related to the autofluorescence of plastic materials[44], as confirmed by the autofluorescence assay
Average concentrations of polystyrene nanoplastic beads (PSN/mL) of 50 and 100 nm detected in the sample used for quality assurance and quality control
PSN size | Sample type | Emission 620 nm (AU) | Initial theoretical concentration | Final measured concentration |
50 nm | Water blanks | NA | - | NA |
Negative controls | NA | - | NA | |
Digestion controls | 5,615 | 8.41 × 1013 | 3.29 × 1010 | |
Biological controls | 2,827 | 8.02 × 1013 | 4.26 × 1010 | |
100 nm | Water blanks | NA | - | NA |
Negative controls | NA | - | NA | |
Digestion controls | 6,683 | 1.15 × 1013 | 1.24 × 1012 | |
Biological controls | 1,792 | 8.35 × 1012 | 4.16 × 1011 |
Detection of PSNPs in biofilms
Samples’ fluorescence at 620 nm was mostly below 5,000 AU and ranged from 320 to 4,800 AU [Supplementary Table 3]. The three samples from the most contaminated station emitted a fluorescence signal above 5,000 at 620 nm (i.e., 5,316, 5,355, and 6,253). All samples’ fluorescence signal fell along the linear part of the
Figure 2. Estimated concentrations of nanoplastic (PSNP/mg DW) in the biofilm samples collected across the 14 stations (× 3 sampling points) visited at Lake St-Pierre. Codes on the X-axis represent station location where A = archipelago, N = north shore, S = south shore. Colors represent water masses, where brown for the northern water body, green for the central water body, and lilac for the southern water body. The solid black line represents the median concentration, and the black dot represents the mean concentration. Stations are presented from upstream to downstream, with station A1 being the most upstream station. Distances in km from station A1 are also indicated. PSNP: Polystyrene-like nanoplastics; DW: dry weight.
Sampling stations were grouped according to their water mass (central, northern, and southern). The central water mass was the least contaminated with PSNPs, where a median concentration of 5.24 × 108 PSNP/mg biofilm DW and an average concentration of 5.01 × 108 ± 3.06 × 108 PSNP/mg biofilm DW were estimated. The southern water mass was the most contaminated, with a median concentration of 3.02 × 109 PSNP/mg biofilm DW and a mean concentration of 3.18 × 109 ± 1.78 × 109 PSNP/mg biofilm DW. As for the northern water mass, it was three times less contaminated than the southern water mass, and twice as contaminated as the central water mass. The median concentration in the northern water mass was 1.03 × 109 PSNP/mg biofilm DW and the average concentration was 1.16 × 109 ± 5.02 × 108 PSNP/mg biofilm DW. A Kruskall-Wallis test performed to assess the difference in PSNP concentrations between the three water masses showed that there was a significant effect of water mass (P < 0.001) [Figure 3]. The Dunn post-hoc test revealed significant differences between central and northern water masses (P = 0.002), southern and central water masses (P < 0.001), and northern and southern water masses (P = 0.015). The epiphyton from the submerged vegetation collected in the southern water mass appeared to have accumulated six times more PSNP/mg biofilm DW than the epiphyton from the central water mass.
Figure 3. Estimated concentrations of nanoplastic (PSNP/mg DW) in the biofilm samples collected across the 14 stations grouped according to their respective water mass (central, northern and southern). The central water body includes three stations (A5, A7 and S1), the northern water body includes nine stations (A1 to A4, A6, A8, A9, N1 and N2), and the southern water body includes two stations (S2 and S3). The solid black line represents the median concentration, and the black dots represent the mean concentration. The letters a, b, and c indicate significant differences in estimated concentrations of nanoplastics between water masses. PSNP: Polystyrene-like nanoplastics; DW: dry weight.
DISCUSSION
Methodological development of the DCVJ probe
Water blanks and digestion controls used in the QAQC assay did not exhibit a fluorescence signal at
Overall, the QAQC assay, in addition to the autofluorescence assay [Supplementary Table 2], demonstrated the method’s ability to detect NPs resembling PSN in the complex epiphyton matrix, and enabled a comparison of NP contamination between stations and between water masses of LSP. However, there might be a marked underestimation of PSNPs detected in biofilm samples, given the loss of PSN used in the QAQC assay. Therefore, the DCVJ probe currently provides information on whether or not epiphythic biofilms can accumulate NPs from LSP and enables comparison between stations but cannot be used for absolute quantification. Further research and method development are needed to improve the quantitative assessment of PSN in periphyton samples, such as studying the interaction between organic matter and the DCVJ probe, and to link periphyton biochemical properties to potential interactions (such as lipid content). The use of the DCVJ probe could be coupled with other analytical methods, such as nanoparticle tracking analysis (NTA) or pyrolysis-GC/MS. These methods also require handling and sample processing, with the potential introduction of other methodological biases.
Developing protocols and methods to properly analyze and quantify NPs is an ongoing process with multiple challenges due to the complex nature of NPs contamination[49,50]. There are currently very few methodologies enabling rapid quantitative analysis of plastic particles, and most of them solely focus on MPs in environmental water samples[51-53], which are less complex than organic matrices such as periphytic biofilms. The DCVJ probe has been successfully applied to quantify PSN in diverse aquatic and terrestrial organisms[35,37,54], demonstrating promise for high-throughput analysis of environmental samples and organisms. The approach allows for effective means of comparison between treatments or sampling stations, although it still needs further development and adjustments to improve quantification depending on the nature and biochemical complexity of the samples being analyzed.
Presence of PSNPs in biofilms
Lower concentrations of PSNPs in the central water mass of LSP compared to the lateral water masses might be linked to the water residence time and, therefore, to the water flow regime. In this zone, the water flow regime is higher, with a residence time of 20 h, while it can reach up to 72 h and even several weeks during the summer low-water period in the lateral water masses[55]. High water flow regime, such as in the central water mass, may promote the dispersal and movements of particles[56] and thus prevent NP accumulation in biofilms. The lower PSNP concentrations observed in the central water mass might also be linked to the origin of the water coming from Lake Ontario, where this lentic environment favors particle sedimentation. Another factor that can drive NP contamination is point sources[57], and direct effluent sources potentially containing plastic pollution may not be as present in this central zone of the lake compared to the lateral water masses. For example, station A5 (lowest estimated PSNP concentration) is surrounded by a protected area[27], which can help limit inputs of plastic debris.
High concentrations of PSNPs observed in the epiphyton collected in lateral water masses might be attributed to the proximity to sources of pollution along the lake shore[58]. This observation is consistent with the results by Crew et al. (2020), who assessed MPs in the sediments of the St. Lawrence River and found a fourfold difference between MP concentrations in sampling points located around the LSP archipelago (n = 3) and the one station located on the north shore of LSP[11]. In lateral water masses, sources of pollution seem mainly linked to agricultural land use and urban areas. In the southern water mass, elevated NP concentrations were mainly driven by station S2, where the average concentration reached
Finally, differences in PSNP concentrations between the northern and southern water masses might reflect the general pollution level of their affluents. For instance, the water quality (i.e., nitrogen, phosphorus, suspended particle matter, and bacteriological loads) is poorer in the tributaries of the south shore compared to those of the north shore[63]. For example, at the St. Lawrence River scale, the annual total suspended particle load between 2013 and 2017 was 1.3 times higher in the tributaries of the south shore than in the tributaries of the north shore[63]. Higher concentrations of all other parameters (i.e., nitrogen, phosphorus and bacteriological loads) were also observed in water samples collected from the south shore than in samples collected from the north shore. For instance, Maskinongé River, which is in close proximity to station A8 in the northern water body, exhibited a better water quality than Yamaska and St-François rivers, which were the main tributaries discharging upstream of the most contaminated station (S2) in the southern water body.
Comparing PSNP concentrations in the biofilm growing on submerged vegetation from LSP with NP concentrations found in other systems around the world is difficult as, to our knowledge, there are no other studies focusing on NPs in this biological compartment. The same goes for other benthic compartments, such as the sediments, as empirical data are lacking due to technical limitations to retrieving NPs particles[64] or analytical limitations to detecting nanoscale particles[57]. However, laboratory experiments highlighted a continuum between MPs and NPs, where MPs subsequently degrade into NPs[3]. For example, Wagner and Lambert (2016) investigated the degradation of different plastic types under aqueous conditions and observed that PS of 1 × 1 cm squares size generated the highest number of particles (6.4 × 108 particles/mL) in the 30-2,000 nm size range[65]. Based on this, the elevated PSNP concentrations observed in the epiphyton of LSP seem plausible, considering the elevated concentrations of MPs found in the sediments of the St. Lawrence River[11].
CONCLUSION
The present study is the first to investigate NPs (resembling polystyrene) contamination in the biofilm growing on the submerged vegetation. The DCVJ fluorescent probe was used to detect and quantify these NPs. This analytical method provided insights into a rapid and cost-effective approach enabling the quantification of PSNPs and laid the foundations for the development of field monitoring protocols in a context where standardized and accessible protocols are lacking. However, the method needs further improvement, particularly in its ability to detect and quantify a broader range of plastic polymers. Moreover, the DCVJ probe can currently only be employed as a diagnostic tool for plastic pollution as the quantified particles need to be correlated with a calibration standard, in this case, PSN. Therefore, it is essential to compare the DCVJ method with other techniques, such as pyrolysis-GC/MS, to obtain absolute quantification and to provide valuable complementary information from a qualitative perspective. Finally, insights into samples’ biochemical composition (e.g., lipids, amino acids) could help to better understand the potential in situ interactions between biofilms and NPs contamination.
Overall, the analysis of biofilm samples collected from submerged vegetation revealed an important accumulation of PSNPs, supporting the hypothesis that periphyton can act as a sink for plastic particles. Estimated levels of PSNPs were linked to the three water masses of LSP with higher concentrations in stations located in lateral waters compared to the central water mass, suggesting an important influence of land-based sources from the tributaries. Further research is needed to better assess the link between specific anthropogenic activities at the watershed scale and NPs pollution.
DECLARATIONS
Authors’ contributions
Field sampling: Mouatchô LY, Ponton DE, Amyot M, Lavoie I
Sample preparation: Mouatchô LY
Laboratory analyses: Roubeau Dumont E
Data analysis: Mouatchô LY
Study design: Lavoie I, Ponton DE, Amyot M, Mouatchô LY
Funding acquisition: Lavoie I, Gagné F, Amyot M
Manuscript writing: Mouatchô LY, Roubeau Dumont E, Lavoie I
Manuscript review and editing: Mouatchô LY, Roubeau Dumont E, Ponton DE, Gagné F, Amyot M, Lavoie I
All authors have read and approved the final manuscript.
Availability of data and materials
The data are available in Supplementary Materials and can also be available from the corresponding author upon reasonable request.
Financial support and sponsorship
This research was supported by a grant from Fonds de recherche du Québec - Nature et technologies (FRQNT) to Lavoie I and fundings from Groupe de Recherche Interuniversitaire en Limnologie (GRIL) provided to Mouatchô LY.
Conflicts of interest
Gagné F is an Editorial Board member of the journal Water Emerging Contaminants & Nanoplastic, while the other authors have declared that they have no conflicts of interest.
Ethical approval and consent to participate
Not applicable.
Consent for publication
Not applicable.
Copyright
© The Author(s) 2024.
Supplementary Materials
REFERENCES
1. Thompson RC, Moore CJ, vom Saal FS, Swan SH. Plastics, the environment and human health: current consensus and future trends. Philos Trans R Soc Lond B Biol Sci 2009;364:2153-66.
2. Plastics Europe. Plastics - the fast facts 2023. Available from: https://plasticseurope.org/knowledge-hub/plastics-the-fast-facts-2023/. [Last accessed on 9 Nov 2024].
3. Ter Halle A, Jeanneau L, Martignac M, et al. Nanoplastic in the North Atlantic subtropical gyre. Environ Sci Technol 2017;51:13689-97.
4. Kalčíková G, Skalar T, Marolt G, Jemec Kokalj A. An environmental concentration of aged microplastics with adsorbed silver significantly affects aquatic organisms. Water Res 2020;175:115644.
5. Granek EF, Brander SM, Holland EB. Microplastics in aquatic organisms: improving understanding and identifying research directions for the next decade. Limnol Oceanogr Lett 2020;5:1-4.
6. Bucci K, Tulio M, Rochman CM. What is known and unknown about the effects of plastic pollution: a meta-analysis and systematic review. Ecol Appl 2020;30:e02044.
7. Laist DW. Impacts of marine debris: entanglement of marine life in marine debris including a comprehensive list of species with entanglement and ingestion records. In: Coe JM, Rogers DB, editors. Marine debris. New York: Springer; 1997. pp. 99-139.
8. Revel M, Roman C, Châtel A. Is cell culture a suitable tool for the evaluation of micro- and nanoplastics ecotoxicity? Ecotoxicology 2021;30:421-30.
9. Campanale C, Dierkes G, Massarelli C, Bagnuolo G, Uricchio VF. A relevant screening of organic contaminants present on freshwater and pre-production microplastics. Toxics 2020;8:100.
10. Peng J, Wang J, Cai L. Current understanding of microplastics in the environment: occurrence, fate, risks, and what we should do. Integr Environ Assess Manag 2017;13:476-82.
11. Crew A, Gregory-Eaves I, Ricciardi A. Distribution, abundance, and diversity of microplastics in the upper St. Lawrence River. Environ Pollut 2020;260:113994.
12. Karlsson TM, Vethaak AD, Almroth BC, et al. Screening for microplastics in sediment, water, marine invertebrates and fish: method development and microplastic accumulation. Mar Pollut Bull 2017;122:403-8.
13. Battin TJ, Besemer K, Bengtsson MM, Romani AM, Packmann AI. The ecology and biogeochemistry of stream biofilms. Nat Rev Microbiol 2016;14:251-63.
14. Kalčíková G, Bundschuh M. Aquatic biofilms-sink or source of microplastics? A critical reflection on current knowledge. Environ Toxicol Chem 2022;41:838-43.
15. Guasch H, Bernal S, Bruno D, et al. Interactions between microplastics and benthic biofilms in fluvial ecosystems: knowledge gaps and future trends. Freshw Sci 2022;41:442-58.
16. Merbt SN, Kroll A, Tamminen M, et al. Influence of microplastics on microbial structure, function, and mechanical properties of stream periphyton. Front Environ Sci 2022;10:928247.
17. Miao L, Hou J, You G, et al. Acute effects of nanoplastics and microplastics on periphytic biofilms depending on particle size, concentration and surface modification. Environ Pollut 2019;255:113300.
18. Wagner M, Lambert S. Freshwater microplastics: emerging environmental contaminants? Springer International Publishing: Cham; 2018.
19. Krause S, Baranov V, Nel HA, et al. Gathering at the top? Environmental controls of microplastic uptake and biomagnification in freshwater food webs. Environ Pollut 2021;268:115750.
20. Wang B, Lan X, Zhang H, Hu Y. Benthic biofilms in riverine systems: a sink for microplastics and the underlying influences. Environ Pollut 2023;337:122607.
21. Chatterjee S, Krolis E, Molenaar R, Claessens MM, Blum C. Nile red staining for nanoplastic quantification: overcoming the challenge of false positive counts due to fluorescent aggregates. Environ Chall 2023;13:100744.
22. Gallitelli L, Cera A, Cesarini G, Pietrelli L, Scalici M. Preliminary indoor evidences of microplastic effects on freshwater benthic macroinvertebrates. Sci Rep 2021;11:720.
23. Osman DM, Yuan W, Shabaka S, et al. The threat of micro/nanoplastic to aquatic plants: current knowledge, gaps, and future perspectives. Aquat Toxicol 2023;265:106771.
24. Triebskorn R, Braunbeck T, Grummt T, et al. Relevance of nano- and microplastics for freshwater ecosystems: a critical review. TrAC Trend Anal Chem 2019;110:375-92.
25. Castaño-Ortiz JM, Romero F, Cojoc L, et al. Accumulation of polyethylene microplastics in river biofilms and effect on the uptake, biotransformation and toxicity of the antimicrobial triclosan. Environ Pollut 2024;344:123369.
26. Holzer M, Mitrano DM, Carles L, Wagner B, Tlili A. Important ecological processes are affected by the accumulation and trophic transfer of nanoplastics in a freshwater periphyton-grazer food chain. Environ Sci Nano 2022;9:2990-3003.
27. MELCCFP. Lake Saint-Pierre, a jewel to be restored. 2013. (in French). Available from: https://belsp.uqtr.ca/id/eprint/421. [Last accessed on 9 Nov 2024].
28. RSIS Lake Saint-Pierre. Ramsar sites information service. (in French). Available from: https://rsis.ramsar.org/fr/ris/949. [Last accessed on 9 Nov 2024].
29. Doucet C, Girard C, Clément-Robert G, Slevan-Tremblay I, Royer Boutin P, Boissonneault Y. Regional integrated management plan for Lake Saint-Pierre. 2018. (in French). Available from: https://belsp.uqtr.ca/id/eprint/1428/1/TCRLSP%201_2018_%20Plan_gestion_int%c3%a9gr%c3%a9e_A.pdf. [Last accessed on 9 Nov 2024].
30. Lalonde S, Cusson B, Longpré D. Current state of knowledge regarding the vegetation on the south shore of Lake Saint-Pierre. 2003. (in French). Available from: https://belsp.uqtr.ca/id/eprint/331/1/Lalonde%20et%20al_2003_vegetation%20rive%20sud%20lac%20St-Pierre_A.pdf. [Last accessed on 9 Nov 2024].
31. Richard G, Côté D, Mingelbier M, Jobin B, Morin J, Brodeur P. Land cover of the Lake Saint-Pierre (St. Lawrence River) floodplain between the years 1950, 1964 and 1997: interpretation of aerial photographs, digitation and preparation of a georeferenced database. (in French). Available from: https://belsp.uqtr.ca/id/eprint/497/. [Last accessed on 9 Nov 2024].
32. TCRLSP. Fact sheet regarding improvements to water quality in Lake Saint-Pierre. 2018. (in French). Available from: https://belsp.uqtr.ca/id/eprint/1432/1/TCRLSP%204_2018_qualit%C3%A9_eau_Fiche%20synth%C3%A8se_A.pdf. [Last accessed on 9 Nov 2024].
33. TCRLSP. Introductory document to the regional integrated management plan for Lake Saint-Pierre, 2018. (in French). Available from: https://belsp.uqtr.ca/id/eprint/1428. [Last accessed on 9 Nov 2024].
34. Restoration of Habitats in Lake Saint-Pierre: a prerequisite for recovery of the Yellow Perch. natcan 2014;138:50-61.
35. Moraz A, Breider F. Detection and quantification of nonlabeled polystyrene nanoparticles using a fluorescent molecular rotor. Anal Chem 2021;93:14976-84.
36. Gagné F. Detection of polystyrene nanoplastics in biological tissues with a fluorescent molecular rotor probe. J Xenobiot 2019;9:8147.
37. Gagné F, Roubeau-Dumont E, André C, Auclair J. Micro and nanoplastic contamination and its effects on freshwater mussels caged in an urban area. J Xenobiot 2023;13:761-74.
38. Description des caractéristiques du lac Saint-Pierre. Source: Ministère de l’Environnement et de la Faune, Lac Saint-Pierre. Fiche d’information sur les zones humides Ramsar, 1998, pp. 1-3. Available from: https://oraprdnt.uqtr.uquebec.ca/portail/gscw031?owa_no_site=665&owa_no_fiche=109. [Last accessed on 9 Nov 2024].
39. Genovese A, Hudon C, Martel AL, Cattaneo A. Molluscan assemblages under multiple stressors in a large fluvial lake. Fund Appl Limnol 2016;188:289-307.
40. Villemure I. I Impact of trophic exposure to a major municipal effluent on the physiological responses of two mobile predator fish species. Theses, University of Quebec in Trois-Rivières: Trois-Rivières, 2015. Available from: https://depot-e.uqtr.ca/id/eprint/7801/1/031118297.pdf. [Last accessed on 9 Nov 2024]
41. Li J, Liu H, Paul Chen J. Microplastics in freshwater systems: a review on occurrence, environmental effects, and methods for microplastics detection. Water Res 2018;137:362-74.
42. Thiele CJ, Hudson MD, Russell AE. Evaluation of existing methods to extract microplastics from bivalve tissue: adapted KOH digestion protocol improves filtration at single-digit pore size. Mar Pollut Bull 2019;142:384-93.
43. Morgana S, Casentini B, Tirelli V, Grasso F, Amalfitano S. Fluorescence-based detection: a review of current and emerging techniques to unveil micro/nanoplastics in environmental samples. TrAC Trend Anal Chem 2024;172:117559.
44. Monteleone A, Brandau L, Schary W, Wenzel F. Using autofluorescence for microplastic detection - Heat treatment increases the autofluorescence of microplastics1. Clin Hemorheol Microcirc 2020;76:473-93.
45. Lee CH, Fang JK. Effects of temperature and particle concentration on aggregation of nanoplastics in freshwater and seawater. Sci Total Environ 2022;817:152562.
46. Peiponen KE, Roussey M. Prediction of nanoplastics aggregation in wastewaters. Water Emerg Contam Nanoplastics 2023;2:12.
47. Pradel A, Catrouillet C, Gigault J. The environmental fate of nanoplastics: what we know and what we need to know about aggregation. NanoImpact 2023;29:100453.
48. Katsumiti A, Losada-Carrillo MP, Barros M, Cajaraville MP. Polystyrene nanoplastics and microplastics can act as Trojan horse carriers of benzo(a)pyrene to mussel hemocytes in vitro. Sci Rep 2021;11:22396.
49. Jakubowicz I, Enebro J, Yarahmadi N. Challenges in the search for nanoplastics in the environment - a critical review from the polymer science perspective. Polym Test 2021;93:106953.
50. Choi S, Lee S, Kim MK, Yu ES, Ryu YS. Challenges and recent analytical advances in micro/nanoplastic detection. Anal Chem 2024;96:8846-54.
51. Huber MJ, Ivleva NP, Booth AM, et al. Physicochemical characterization and quantification of nanoplastics: applicability, limitations and complementarity of batch and fractionation methods. Anal Bioanal Chem 2023;415:3007-31.
52. Okoffo ED, Thomas KV. Quantitative analysis of nanoplastics in environmental and potable waters by pyrolysis-gas chromatography-mass spectrometry. J Hazard Mater 2024;464:133013.
53. Wang X, Li Y, Kroll A, Mitrano DM. Differentiating microplastics from natural particles in aqueous suspensions using flow cytometry with machine learning. Environ Sci Technol 2024;58:10240-51.
54. Chakraborty N, Silswal A, Koner AL. Julolidine-based fluorescent molecular rotor: a versatile tool for sensing and diagnosis. Sens Diagn 2024;3:585-98.
55. Comité ZIP of Lake Saint-Pierre Summary sheet on improving water quality in Lake Saint-Pierre. 2018. (in French). Available from: https://belsp.uqtr.ca/id/eprint/1432/1/TCRLSP%204_2018_qualit%C3%A9_eau_Fiche%20synth%C3%A8se_A.pdf. [Last accessed on 9 Nov 2024].
56. He B, Smith M, Egodawatta P, Ayoko GA, Rintoul L, Goonetilleke A. Dispersal and transport of microplastics in river sediments. Environ Pollut 2021;279:116884.
57. Hale RC, Seeley ME, La Guardia MJ, Mai L, Zeng EY. A global perspective on microplastics. JGR Oceans 2020;125:e2018JC014719.
58. Eerkes-Medrano D, Thompson RC, Aldridge DC. Microplastics in freshwater systems: a review of the emerging threats, identification of knowledge gaps and prioritisation of research needs. Water Res 2015;75:63-82.
59. COGESAF. Water master plan for the Saint-François River watershed. 2014. (in French). Available from: https://cogesaf.qc.ca/PDE/COGESAF_PDE_2014-2023.pdf. [Last accessed on 9 Nov 2024].
60. MRC. Haute Yamaska Revised water master plan (2017-2021) - For healthy lakes and streams in Haute-Yamaska. 2017. (in French). Available from: https://haute-yamaska.ca/wp-content/uploads/2018/08/PDE-version-finale_3-mai-2018-web.pdf. [Last accessed on 9 Nov 2024].
61. Granby. Industrial business directory. (in French). Available from: https://granby-industriel.com/entreprises/. [Last accessed on 9 Nov 2024].
62. MRC. Maskinongé Revised land use and development plan. Plan 9.9C - Maskinongé: Lake Saint-Pierre shoreline zoning. 2022. (in French). Available from: https://mrcmaskinonge.ca/wp-content/uploads/schema-amenagement-plan-9-9c.pdf?v=1620672520. [Last accessed on 9 Nov 2024].
63. MELCCFP. Loadings of six physicochemical and bacteriological parameters at the mouth of the main tributaries of the St. Lawrence River - 2013-2017. 2024. (in French). Available from: https://www.environnement.gouv.qc.ca/Eau/flrivlac/physicochimie-bacteriologie/rapport-charges-six-paramettres-physicochimiques-bacteriologique.pdf. [Last accessed on 9 Nov 2024].
64. Uddin S, Fowler SW, Habibi N, Behbehani M. Micro-nano plastic in the aquatic environment: methodological problems and challenges. Animals 2022;12:297.
Cite This Article

How to Cite
Mouatchô, L. Y.; Roubeau Dumont, E.; Ponton, D. E.; Gagné F.; Amyot, M.; Lavoie, I. Use of a fluorescent molecular rotor probe for nanoplastics assessment in epiphytic biofilms growing on submerged vegetation of Lake Saint Pierre (St. Lawrence River). Water Emerg. Contam. Nanoplastics. 2024, 3, 22. http://dx.doi.org/10.20517/wecn.2024.39
Download Citation
Export Citation File:
Type of Import
Tips on Downloading Citation
Citation Manager File Format
Type of Import
Direct Import: When the Direct Import option is selected (the default state), a dialogue box will give you the option to Save or Open the downloaded citation data. Choosing Open will either launch your citation manager or give you a choice of applications with which to use the metadata. The Save option saves the file locally for later use.
Indirect Import: When the Indirect Import option is selected, the metadata is displayed and may be copied and pasted as needed.
About This Article
Copyright
Data & Comments
Data
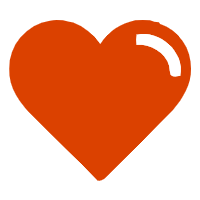
Comments
Comments must be written in English. Spam, offensive content, impersonation, and private information will not be permitted. If any comment is reported and identified as inappropriate content by OAE staff, the comment will be removed without notice. If you have any queries or need any help, please contact us at support@oaepublish.com.