First, do no harm: the role of preclinical animal models in predicting adverse events in gene therapy clinical trials for Duchenne muscular dystrophy and X-Linked myotubular myopathy
Abstract
The occurrence of severe adverse events (SAEs) in patients with Duchenne muscular dystrophy (DMD), X-linked myotubular myopathy (XLMTM), and other neuromuscular diseases treated with adeno-associated virus (AAV) constructs has prompted studies to improve the safety and efficacy of gene therapy. Physicians have weighed the medical tenet of “first, do no harm” against the perspective of patients with progressive life-threatening conditions who may accept greater risk. Regarding SAE pathogenesis, discussion has focused on total AAV exposure and patient mutations more likely to induce immunity, while stressing the limitations of animal models in predicting adverse events. Therapeutic strategies for reducing side effects have employed more myotropic AAV serotypes and efficient transgenes. Other recommendations include excluding certain DMD gene mutations associated with SAEs and substituting less immunogenic transgenes such as utrophin (DMD) and myotubularin-related protein (XLMTM). For the sake of preclinical studies, emphasis has been placed on outbred rodents and larger animals that better predict immunity. Here, the absence of side effects in canine DMD and XLMTM models might be explained partly by phenotypic differences between affected humans and dogs. Specifically, dystrophin- and myotubularin-deficient dogs exhibit milder lesions, including less muscle fat deposition and the absence of hepatopathy, respectively, which could lead to reduced immune responses to AAV constructs. To better predict future problems, thought should be given to tracking early subclinical markers of the innate immune response, especially complement activation. Regardless of steps taken to improve the predictive value of animal models for SAEs, some questions will only be answered through human clinical trials after carefully considering the risk-benefit ratio.
Keywords
INTRODUCTION
The long-held promise of gene therapy to improve the lives of individuals with devastating diseases is finally being realized, with over 2,700 studies currently listed on the ClinicalTrials.gov website. This includes a variety of gene therapy products, like plasmid DNA, gene editing technology, viral vectors, and stem cells. Among viral vectors, adeno-associated viruses (AAVs), adenoviruses, and lentiviruses are used most, with AAVs predominating[1]. The original appeal of AAVs was based on their ability to achieve widespread gene transfer at clinically relevant doses in mice, dogs, and nonhuman primates (NHPs)[2]. However, severe adverse events (SAEs), not predicted by preclinical studies, have recently been seen in Duchenne muscular dystrophy (DMD) and X-linked myotubular myopathy (XLMTM) patients after AAV-transgene therapy[2-8]. Similar SAEs have been reported with AAV therapy in spinal muscular atrophy[5-9], as well as Pompe[5-9] and Danon[5] glycogen storage diseases. Taken together, this has raised questions about the ability of canine and other animal models to predict SAEs and suggests that alternative strategies may be needed.
HIPPOCRATIC OATH AND ETHICAL CONSIDERATIONS
The ethical standard “first (above all), do no harm,” from the Latin primum non nocere, has guided physicians in treating patients since antiquity[10,11]. Usually attributed to the Greek physician Hippocrates and associated with the Oath bearing his name, the phrase’s exact origins and meaning are controversial due to nuances of translation, shortcomings of historical accounts, and the changing moral values of society[12,13]. While the precise wording, “first, do no harm,” is not included in the original Hippocratic Oath, the phrase can be inferred from other wording and additional writings by Hippocrates and his students during this period[11].
Regardless of the exact phrase used and to whom the expression is attributed, a distinction must be made between the potential for side effects of any treatment and those caused by negligence (so-called injustice or non-maleficence)[11]. This is especially relevant with devastating diseases for which a higher risk-benefit ratio could logically be tolerated. In this context, the Oath is seen by some as paternalistic without sufficient regard given to the perspective and autonomy of patients and their parents[10,14]. Indeed, surveys show that Duchenne patients may be more willing to accept a higher risk of SAEs than their treating clinicians[6]. This is an example of “shared decision making” employed in cancer treatment[15], wherein patients accept greater risks while physicians focus more on the possibility of adverse events[16].
Finally, from an ethical standpoint, there are concerns regarding the limited access of older, non-ambulatory DMD patients to any impactful treatment, especially gene therapy, despite potential benefits for upper limb and cardiopulmonary function[17,18]. This situation is troubling because both the relative risks and benefits of genetic treatments are typically worsened by advancing age. To ensure that the needs of these boys and young men with DMD are adequately addressed, it is essential to continuously update outcome information for patients, families, and physicians. One approach could involve creating a database of anonymized patient ages, interventions, and outcomes, initially available to physicians at specialty centers of excellence, where the highest-risk, oldest patients are most likely to seek evaluation for treatment. Only with access to this granular information can physicians maintain the standard of primun non nocere in the era of extended indications for complex biologics to treat lethal diseases (see the FDA’s recent approval of Sarepta’s microdystrophin Elevidys for non-ambulatory patients below).
The experiences of all five authors have shaped this review. Stedman and Byrne addressed the ethical principles surrounding gene therapy in 2012, coinciding with the initial clinical trials of AAV-microdystrophin constructs for DMD[19]. These ethical considerations are arguably even more relevant today. Back in 2012, the potential for destructive immune-mediated myositis induced by locally or regionally administered compounds was projected as an important ethical dilemma. To justify safely moving forward with further human trials, the article referenced the ability of dogs to predict immunity in other diseases, such as hemophilia, a field to which Dr. Stedman has made significant contributions[20]. Reflecting his own support for using dogs to test efficacy and predict immunogenicity in gene therapy, Dr. Byrne has worked to develop a canine model of Pompe disease[21]. In their 2012 article, they concluded, “A convincing demonstration of durable efficacy following vascular delivery in a canine model would be reassuring”.
Ultimately, the Kornegay laboratory[22] and others[23,24] have achieved sustained microdystrophin expression without toxicity in dystrophic dogs treated systemically with AAV vectors carrying a canine transgene. However, DMD patients treated with the same construct containing a human transgene at comparable doses experienced SAEs (as discussed below). A similar scenario occurred in XLMTM preclinical studies conducted by Childers and his colleagues, where myotubularin (MTM1)-deficient dogs treated with an AAV construct expressing the canine MTM1 gene had remarkable improvement without toxicity[25], whereas some XLMTM patients developed fatal SAEs when the human transgene was used[26].
Dr. Michael Lawlor has assessed humans with DMD and XLMTM as well as genetically homologous animal models treated with AAV-transgenes[22,25-30]. He brings an essentially unique perspective on the phenotypic differences across these conditions, including the relative degrees of acute necrosis vs. chronic fibrosis and fatty deposition in DMD, as well as liver involvement in XLMTM. These microscopic changes, along with the variable underlying mutations, offer the most useful clues to explain SAEs seen in humans but not animal models[28,31].
SEVERE ADVERSE EVENTS (SAES) AND AAV-BASED CLINICAL TRIALS
Several recent reviews have described SAEs, including hepatotoxicity, thrombocytopenia, thrombotic microangiopathy (TMA), atypical hemolytic uremia syndrome (aHUS), myocarditis, and myositis,[2-7,26,31] linked to innate and adaptive immune responses as well as complement activation[32,33] in AAV-transgene clinical trials [Table 1]. The DMD trials used a miniaturized microdystrophin version of the DMD gene with portions of the rod domain removed to fit the limited AAV carrying capacity[3,5]. A full-length version of the MTM1 gene was used in the XLMTM trial[26]. Similar adverse effects have been characterized in patients receiving AAV-transgene products for other neuromuscular diseases such as spinal muscular atrophy[5-9] and Pompe[5-9] and Danon[5] glycogen storage diseases. Less frequent systemic or ocular SAEs have been observed in hemophilia[7,34], lysosomal storage diseases (LSDs)[3], and degenerative retinal disorders[35], for which canine models have been used to demonstrate treatment efficacy and side effects[20,36-44].
Adverse events in AAV-based neuromuscular disease clinical trials*
Disease | Company (product/transgene) | AAV serotype | Highest AAV dose (vg/kg) | Adverse events | *Additional references |
DMD | Sarepta (elandistrogene moxeparvovec) | AAVrh74 | 1.33E14 | Vomiting, liver injury with increased transaminases, rhabdomyolysis, myositis, myocarditis | Mendell et al., 2023[176] |
DMD | Solid Biosciences (SGT-101) | AAV9 | 2E14 | Thrombocytopenia, renal damage, cardiopulmonary insufficiency, myocarditis | Dreghici et al., 2024[177] |
DMD | Pfizer (fordadistrogene movaparvovec) | AAV9 | 2E14 | Thrombocytopenia, aHUS/thrombotic microangiopathy, myocarditis | Pfizer press release, 2024[178] |
XLMTM | Astellas (resamirigene bilparvovec) | AAV8 | 3.5E14 | Hepatic toxicity with hyperbilirubinemia, sepsis | Shieh et al., 2023[26] |
SMA | Novartis (onasemnogene abeparvovec) | AAV9 | 1.1E14 | Fever, malaise, vomiting, acute liver failure, thrombocytopenia | Chand et al., 2021[9] |
Cellular and humoral immunity to either the AAV vector or transgene product act together to induce these side effects[45] [Figure 1]. A range of risk factors could be involved, including the antigenicity of AAV capsid proteins and other construct components, the patient’s genetic mutation, prior exposure to AAV and overall immune status, and product impurities resulting from the AAV manufacturing process[46]. Perhaps most importantly, the incidence of side effects has tended to correlate directly with AAV vector genome (vg) dose. Treatment effect has generally required dosing at 1-3E14[47], while toxicities in DMD and XLMTM have mostly occurred at or above a dosage of 1.1E14 vg/kg[4,33]. Obviously, there is a small therapeutic window that likely varies among patients.
Figure 1. Schematic of four quadrants in which cellular and humoral immunity interact with AAV capsid and transgene proteins to cause SAEs. From Hamilton et al., 2021[45]. Republished under Creative Commons Attribution License (CC-BY 3.0). AAV: Adeno-associated virus; SAEs: severe adverse events.
Adaptive immunity against the transgene protein products also plays a role in DMD immunity. A collaborative study of patients with T cell-mediated reactions identified overlapping mutations in the dystrophin amino terminus region[4,31]. Exons 8 to 11 were deleted in all patient DMD genes, presumably causing the protein epitopes translated from the construct to be recognized as non-self-neoantigens [Figure 2] (see discussion of specific microdystrophin constructs below). This harkens back to immunity against dystrophin identified by Mendell et al. in their early AAV-microdystrophin DMD clinical trial[48].
Figure 2. Diagram depicting DMD gene mutations associated with SAEs. Panels A, B, and C show the dystrophin structure, microdystrophin exon content of therapeutic transgenes, and patient mutations, respectively. From Bönnemann et al., 2023[31]. Republished under the Rights Link for Scientific Communications through the Copyright Clearance Center. SAEs: Severe adverse events; DMD: Duchenne muscular dystrophy.
Considering the role of complement in SAEs, markers should be monitored to track activation and response to treatment. In a study of TMA patients with SAEs after systemic AAV administration, immunoglobulin M (IgM) and IgG increased rapidly with an associated rise in D-dimer and a decline in the platelet count[33]. Activation of both classical and alternative complement pathways, as indicated by depleted C4 and elevated soluble C5b-9, Ba, and Bb antigens, occurred.
USING ANIMAL MODELS TO PREDICT TREATMENT SAFETY
The requirement that animals be used to demonstrate the safety (or toxicity) of new drugs dates to the Federal Food, Drug, and Cosmetic Act of 1938. Various regulations were ultimately developed by the US Food and Drug Administration (FDA), with guidelines by and large dictating that safety be demonstrated in two species, a rodent and non-rodent (often the dog), in both short- and long-term studies[49,50]. Recent reviews have suggested that only approximately 50% of animal studies, extending across species including dogs, predict drug toxicity[51]. Related to this observation and broader animal welfare concerns, the FDA Modernization Act 2.0 passed by the US Congress in 2022 removed the requirement for animal use in drug approval and stipulated that alternatives would be allowed[52,53]. This is in keeping with the 3Rs (replacement, reduction, and refinement) approach toward the use of animals in research and could lead to greater reliance on alternatives like computational models, the use of recombinant antibodies produced in cell culture vs. live animals, and tissue engineering (organ on chip) technologies[54]. With that being said, pharmaceutical companies can still use animals to establish safety, and change is expected to occur gradually.
As shown by the SAEs in recent human trials, side effects associated with cell and gene therapy are generally more severe than those caused by traditional drugs. In their 2015 paper, Gopinath et al. emphasized the value of animal models in assessing variables of safety, efficacy, dosage, and localization of gene expression with viral vectors[55]. They stressed that choosing a suitable disease-specific model is of paramount importance for successful clinical translation. Consistent with this approach, the FDA has developed preclinical industry guidelines for cell and gene therapies, recommending 1) in vitro testing (e.g., functional assays, immunophenotyping, and morphologic evaluation) and in vivo pilot studies to establish the biological relevance of a specific animal species to the investigational product(s) before conducting definitive preclinical trials and 2) detailed assessment of the relevancy of each animal species used to support a potential clinical trial, with a summary of this assessment submitted as part of the preclinical section of the Investigational New Drug (IND) application[56-58]. Importantly, general adherence to these guidelines has not prevented recent SAEs in AAV clinical trials.
THE ROLE OF ANIMAL MODELS IN ASSESSING IMMUNITY TO AAV CONSTRUCTS
General considerations
The FDA guidelines mentioned above emphasize the importance of considering species-related immunogenicity in gene therapy trials. Mice used for testing are often inbred with essentially identical genetic makeup. This contrasts with the genetic diversity among humans[59] and likely contributes to the limited ability of inbred mice to predict adverse effects of foreign and/or self-antigens[60]. On the other hand, large animals are reasonably outbred and mostly share physiological functions such as the immune system with humans[61]. In addition, they live comparatively longer, allowing for extended studies, and are similar in size to a neonate or small child so that scaling issues can be addressed. Important limitations include the relatively few well-defined genetic conditions in most large animal species, the high costs of housing and maintenance, difficulties in achieving adequate sample sizes due to longer gestation periods and smaller litter sizes, and concerns about animal welfare[62,63].
Dogs have been used to model a range of human diseases, from cancer[64] to genetic disorders[65]. Beyond the advantages offered by other large animal models, mutations have been identified in an increasing number of inherited diseases, allowing genetic therapies to be assessed[66]. Because pet dogs are treated as family members, sophisticated tests such as magnetic resonance imaging (MRI) and electrodiagnostic procedures routinely used in a veterinary specialty setting can be extrapolated to research models[67,68]. As an added bonus discussed further below, treatments developed in canine models are now benefitting companion dogs[69,70].
Regarding pre-existing immunity to AAV in dogs, one study noted particularly high neutralizing antibody titers to AAV6 in puppies, with levels then tapering[71]. Titers to AAV8 and 9 were less pronounced. Dystrophic dogs mounted a more robust antibody response to AAV, potentially because weaker suckling may have reduced immunoglobulin-induced immunity. Because AAV is a parvovirus, there has been interest in the effect of vaccination routinely employed against this virus in young dogs. Parvovirus vaccination status did not affect the AAV response. Another paper confirmed the selective increase in neutralizing antibodies to AAV6 and AAV1 in dogs[72]. Horses showed a particular increase in antibodies to AAV5, whereas pigs exhibited elevated levels of antibodies to several serotypes. Confirming the clinical significance of these antibodies, AAV transduction was much reduced in mice injected with serum from animals with high AAV titers.
In certain disease settings, dogs have predicted immunotoxicity better than mice and, in some cases, even more effectively than NHPs. The best example is hemophilia, for which naturally occurring models of factor VIII (hemophilia A) and IX (hemophilia B) have been studied for over 70 years[20,36-38]. Studies in canine hemophilia foresaw immunity to adenoviral vectors[37] and increases in transaminase enzymes subsequent to the administration of liver-directed AAV constructs[7]. Hepatocyte clonal expansion associated with AAV genomic DNA integration events after intravenous liver-directed therapy in hemophiliac dogs has raised concerns about long-term supraphysiologic factor levels and hypercoagulability[38]. Further supporting canine hemophilia as a model, companion hemophiliac dogs managed clinically with AAV-factor constructs have had occasional complications analogous to those in humans[69].
Several human clinical trials have utilized AAV-transgene constructs to correct inborn errors of metabolism that cause storage products to accumulate either systemically or predominantly in the central nervous system (CNS)[3]. As briefly mentioned earlier, viral vectors have also been used to treat humans with degenerative retinal diseases, wherein more limited ocular SAEs, often related to surgery instead of an immunologic reaction, have been reported[35]. Adverse events in LSD studies have not been well characterized, making it difficult to define the role animal models could play in their identification. Nevertheless, dogs with spontaneous LSDs[39-41] and degenerative retinal conditions[42-44] have been used to establish the therapeutic value of AAV-transgene constructs in advance of human trials. The construct has typically been given by intrathecal/intracerebral or intraocular administration, respectively, so inflammatory reactions would logically be localized. Adverse events related to the construct have not been reported in the LSD studies. Uveitis occurred after subretinal injection of an AAV-RPE65 construct in a canine Leber congenital amaurosis model[42].
Providing further support for the value of canine models to characterize post-treatment immunity, complement activation has been seen in hemophiliac dogs[73] and in canine models of hypersensitivity reactions[74]. A C5b-9 ELISA assay was used to test hemophiliac dogs. We saw hypersensitivity reactions in golden retriever muscular dystrophy (GRMD) dogs given Nemo Binding Domain (NBD) peptide to inhibit NF-κB but did not assess complement[75].
Preclinical studies for DMD and XLMTM
AAV-transgene constructs
Preclinical studies of AAV-microdystrophin constructs have followed a logical course from both a species and site of delivery perspective. As discussed in our 2012 review of the role of canine models in DMD[67], experiments were done initially in mdx mice[76,77], followed by dystrophic dogs[78-84]. Although there was a minimal immune response in mice, immunotoxicity was evident in dogs. Pioneering research at the University of Washington and Fred Hutchinson Cancer Center in Seattle, led by Jeff Chamberlain, Stephen Tapscott, and Rainer Storb, determined that intramuscular injection of AAV2 and AAV6-microdystrophin constructs elicited a marked cytotoxic T cell response to capsid proteins[78,79]. Studies by the Takeda laboratory in Japan involving beagle dogs carrying the GRMD mutation, injected with an AAV2-CMVLacZ construct, showed an immune response against the β-galactosidase protein, suggesting that dystrophin could act as a neoantigen[80]. Indeed, non-immunosuppressed GRMD dogs treated with an AAV construct containing a codon-optimized human microdystrophin by the Kornegay laboratory developed marked myositis [Figure 3][81] that was not seen in subsequent studies using the same canine gene[82]. This suggested divergent amino acid sequences between species could lead to immunity. Other studies revealed that the immune response to AAV-microdystrophin constructs in dystrophic dogs was lessened by immunosuppression[80,83] and largely absent even without immunosuppression when a canine AAV-microdystrophin and muscle-specific promoter were used[84]. Results from contemporaneous phase 1 human trials of AAV-microdystrophin constructs injected intramuscularly were mixed, with one having no evidence of immunity[85], whereas another had a dystrophin-specific T cell response[48].
Figure 3. T2-weighted magnetic resonance images of pelvic limb muscles 8 weeks after AAV9-CMV-mini-dystrophin vector intravenous injection of two GRMD dogs (A-D, E-H) at 4 days of age. Images were segmented and color-coded to outline individual muscles. Signal-intense lesions are particularly pronounced in the vastus heads of the quadriceps and adductor muscles. These changes persisted with fat saturation, suggesting that they most likely represent fluid due to inflammation or edema. From Kornegay et al., 2010[81]. Republished under Creative Commons Attribution License (CC-BY 3.0).
Based on generally encouraging results of intramuscular delivery of AAV-microdystrophin constructs in dogs, work was extended to high-pressure transvenular regional limb delivery using the Bier block approach applied clinically for local anesthesia and pain relief in the distal limbs[86,87]. Hansell Stedman and his colleagues at the University of Pennsylvania had previously utilized this technique to deliver AAV-factor IX transgene constructs to hemophiliac dogs based on the premise that the factor produced in muscle would move systemically to correct the clotting defect[88]. Striking dystrophin expression was found in dogs using this regional limb delivery technique without immunosuppression, showing more broadly the feasibility of vascular delivery[24,67,89]. The relative absence of an immune response suggested venous injection might actually be less immunogenic, presumably because muscle injury was avoided and antigen was less concentrated. This regional limb technique was then used to safely deliver saline to adult muscular dystrophy patients by colleagues at the University of North Carolina-Chapel Hill[90]. The next logical step would have been to apply the approach in DMD. However, with encouraging results already being established for generalized intravenous delivery of AAV-microdystrophin constructs in dystrophic mice[91,92] and dogs[22-24], research moved towards systemic administration. Canine studies have provided guidance for dosing human AAV constructs. For instance, our GRMD AAV9-microdystrophin study included 1E13, 1E14, and 2E14 vg/kg dosage groups[22], while DMD patients in the Solid Biosciences SGT-001 trial were treated at 5E13 and 2E14 vg/kg.
There is not a naturally occurring dystrophin-deficient NHP model, but CRISPR/Cas9 has been used to disrupt the DMD gene in rhesus monkeys[93] and other species including pigs, rabbits, and rats[94]. Mechanistic studies at the cellular level were done in monkeys, potentially providing a platform for treatment development[95]. Importantly, given that mosaic mutations are generated with CRISPR, care must be taken in interpreting findings[94]. A systematic histopathologic natural history study of dystrophic monkeys would be necessary to determine whether this model is phenotypically analogous to DMD, and the associated likelihood preclinical studies will translate to patients.
Work has also been done in normal NHPs to establish proof-of-concept. As an example, encouraging results were obtained using regional limb delivery of AAV-microdystrophin constructs in cynomolgus macaques[96]. In addition, plasmapheresis lowered anti-AAV antibodies in NHPs with pre-existing AAV immunity[97]. Moreover, there has been natural interest in using monkeys to predict immunity. Dr. Wilson’s lab at the University of Pennsylvania identified sensory neuron/dorsal root ganglia toxicity in pigs and hepatotoxicity in NHPs receiving a dose of 2E14 AAV9-human survival of motor neuron (SMN) protein[98]. A more recent NHP study from his lab detected hepatic microvasculature toxicity after systemic administration of AAV-enhanced green fluorescent protein constructs at doses ≥ 1E14 vg/kg[99]
Regarding preclinical XLMTM studies, MTM1 knockout mice had robust clinicopathologic improvement over a 6-month period after a single tail vein dose of 1E13 AAV8-MTM1[100]. Comparable improvement was seen in a subsequent canine XLMTM study, in which AAV8cMTM1 was dosed in three groups (n = 3/each; 0.3E14, 2E14, and 5E14 vg/kg). Results were compared to those from six untreated controls. Dogs were followed for 17 weeks post-infusion, at which time all untreated controls had died.
Cell-based therapies
As emphasized recently by Graves and Storb[101], “It is difficult to imagine the successful development of hematopoietic cell transplantation (HCT) without the significant contributions made by the canine preclinical animal model.” Studies in normal outbred dogs featured prominently in several advancements, including the importance of histocompatibility matching, establishing long-term donor hematopoietic cell engraftment, prevention of graft-versus-host disease, advancing effective conditioning and post-grafting immunosuppression protocols, and applying HCT to induce tolerance for solid organ and composite tissue transplantation[101,102]. Encouragingly, similar HCT protocols are now being used to manage companion dogs with hematological disorders and lymphoproliferative malignancies[101-103].
Based on promising results in the late ‘90s of HCT in mdx mice[104] and a single DMD patient treated for concomitant severe combined immune deficiency[105], there was keen interest in using HCT in DMD. Given that much of the pioneering canine work on HCT was done at the Fred Hutchinson Cancer Research Center, GRMD/CXMD carriers from the University of Missouri and Cornell were used to establish a colony in Seattle. However, in a subsequent study, dystrophic dogs with allogenic bone marrow engraftment did not have increased dystrophin-positive fibers, wild-type dystrophin RNA, or donor-derived myonuclei[106].
This scenario whereby canine studies did not ultimately confirm findings from mdx mice had precedent from myoblast transplantation studies done in the 1980s and ‘90s. Based on proof-of-principle studies in mdx mice[107], human trials were instituted with disappointing results[108,109]. We had characterized canine myoblasts[110] and conducted analogous transplantation studies in GRMD dogs with little success, turning instead to an autologous cell model preceded by muscle injury[67,111]. Failed translation from mice to humans (and dogs) probably related to variables influenced by scale, such as cell migration[112] and the combined effects of poor blood supply and immune rejection[113,114].
Why were SAEs absent in canine DMD and XLMTM models?
Considering dogs have predicted the outcome of gene and cellular therapy in other settings, why did genomically homologous canine models not identify SAEs seen in DMD and XLMTM clinical trials using comparable AAV doses? Immunity to either the transgene product (dystrophin or myotubularin) or AAV vector could be involved.
The GRMD model has a single base change in the 3’ consensus splice site of intron 6, causing exon 7 to be skipped and termination of the dystrophin reading frame in exon 8[115]. Alternative splicing can restore the reading frame, leading to revertant fibers that could protect against anti-dystrophin immunity[116]. Thus, GRMD dogs may not be prone to the immune reaction to dystrophin evident in DMD boys with mutations in the N-terminal region[31]. Interestingly, the null mutation in the dystrophic German shorthaired pointer model does not allow for alternative spicing and might better predict an immune response against dystrophin (see discussion of the immune response to dystrophin and utrophin in the pointer model below)[117,118].
An analogous situation could exist in XLMTM. Truncating mutations in the human MTM1 gene are associated with a more severe form of the disease compared to non-truncating inframe deletions/insertions and missense mutations[119-121]. Affected dogs have a missense mutation[27], allowing protein production that could establish tolerance. Moreover, as with utrophin in DMD (see below), AAV constructs carrying MTM-related protein (MTMR) transgenes have therapeutic benefits in XLMTM[122,123]. However, mutation type did not appear to contribute to the incidence of SAEs in the XLMTM ASPIRO trial, with no disproportionate increase in myotubularin antibodies seen in patients with null mutations[26].
To ensure that findings from a genomically homologous animal model are optimally translatable to humans, the model must be phenotypically analogous[124]. While both GRMD and canine XLMTM express disease phenotypes similar to those of their human disease counterparts, they are not completely analogous[125] [Figure 4]. One key difference relates to the chronology of disease embodied within the relative longevity of dogs and humans. Based on an epidemiological study of dogs admitted to veterinary medical teaching hospitals, year one of a golden retriever’s life is roughly equivalent to the first 20 years for a human[126]. For the sake of clinical extrapolation, we have divided these periods into four age groups, with 3, 6, 9, and 12 months in GRMD corresponding to 5, 10, 15, and 20 years in DMD[127]. The GRMD and DMD disease phenotypes generally parallel one another for the first 6 months and 10 years, respectively, with each having marked clinicopathologic progression. After 6 months, clinical signs and histopathologic lesions [Figure 5] in GRMD tend to stabilize. Affected dogs often live well into adulthood, whereas DMD patients continue to deteriorate[67,125,127]. Progressive histopathologic disease and associated inflammation in DMD patients[128,129] receiving AAV constructs could predispose them to complement activation and associated SAEs[6]. One particular distinguishing histopathologic feature between DMD and GRMD is the degree of fatty deposition, with affected dogs having markedly less intramuscular fat than humans based on both histopathologic[130,131] [Figures 5 and 6] and MRI assessment[132,133]. Given the role that cytokines secreted by adipocytes (adipokines) play in inflammation[134,135], greater fatty infiltration in DMD boys might predispose them to a more marked immune response.
Figure 4. Comparative XLMTM histopathologic lesions in a MTM1 knockout mouse (43 days, mid-stage), dog (18 weeks, end-stage), and boy (2 months, terminal-stage). Increased numbers of central nuclei (inset in human sample) and fiber size variation due to numerous hypotrophic and atrophic (human) fibers are seen on H&E and PAS stains. Organelle (NADH) and glycogen (PAS) mislocalization is evident as either peripheral halos (human) or dark peripheral staining (dog and mouse). “Necklace fibers” (arrows) are seen in canine, some human patient biopsies (NADH inset), and rare mouse fibers. Scale bar = 40 µm. From Lawlor et al., 2016[28]. Republished under Creative Commons Attribution License (CC-BY 3.0). XLMTM: X-linked myotubular myopathy.
Figure 5. Photomicrographs of H&E (A, B, E, and F) and acidic ATPase (pH 4.3) (C, D, G, and H) stains of the vastus lateralis muscle of GRMD vs. normal dogs at 3 and 12 months, roughly equivalent to 5 and 20 human years based on comparative longevity studies[126], showing relative stabilization of histopathologic lesions, with minimal fatty deposition in GRMD. Note also the increase in type I (darker stained) fibers with some grouping in GRMD. Although there is minimal open space suggestive of fat in GRMD at 12 months, the endomysial connective tissue separating individual myofibers is increased (see Fan et al., 2014[132] for GRMD images at 6 months and Figure 6 for comparison to DMD). Courtesy of Drs. Jane Fan and Yael Shiloh-Malawsky, UNC-Chapel Hill.
Figure 6. Photomicrographs of (A) H&E, (B) GT staining for collagen and connective tissue, (C) Dys2 dystrophin, and (D) utrophin histochemical stains of the vastus lateralis muscle from a 13-year-old boy roughly equivalent to an 8-month-old dog based on comparative longevity studies[126]. In the H&E (A) and GT (B) stains, the endomysial connective tissue separating individual myofibers and open spaces typical of adipose tissue (fat; top right) are increased (see Figure 5 for GRMD comparison). Note also the small cluster of dystrophin-positive (“revertant”) fibers in C (arrow) and prominent utrophin staining consistent with upregulation. Otherwise, there is variation of myofiber size with occasional internal nuclei, both nonspecific features of myopathy, with little to no necrosis or inflammation. Scale bar = 50 µm. GT: Gomori trichrome.
The clinical course of XLMTM in Labrador retrievers is more rapid than in GRMD, consistently causing progressive weakness and muscle atrophy, necessitating euthanasia by 4-6 months of age[125,136]. Nonetheless, considering XLMTM in dogs is caused by a missense mutation[27], the phenotype is likely comparatively milder than in affected humans with truncating mutations. This is substantiated by the 4- to 6-month course of XLMTM in Labradors compared to the rapid deterioration of human infants over a period of only days to weeks[120,137]. More importantly, dogs do not develop cholestatic hepatobiliary disease that seemingly predisposes human XLMTM patients to SAEs[26,138-140].
In light of their historical use to demonstrate efficacy and immunogenicity with gene and cell therapy and as evidenced by recent papers[141,142], canine models will probably continue to be used in AAV-transgene preclinical testing. To better predict future problems, thought should be given to tracking early subclinical markers of the innate immune response, especially complement activation. As precedent, complement C8 alpha chain and several other serum proteins were elevated in subclinical leishmaniasis in dogs and tracked with disease progression[143].
Background on AAV-microdystrophin constructs in DMD clinical trials [Figure 2]
The Chamberlain laboratory has systematically studied structural modifications of the dystrophin central rod domain to identify microdystrophins with maximal functional efficacy[144]. They chose a particular design, named µDys5, for further study because of its functional performance and small size. These studies have been augmented by work from Stephen Hauschka on regulatory cassettes showing that the CK8e cassette consistently drives more muscle-restricted protein expression[145]. Ultimately, µDys5 was coupled with CK8e for clinical development as SGT-001 in collaboration with Solid Biosciences (NCT03368742).
The improved function seen with the CK8e-μDys5 construct can be attributed to several factors[144] (Jeff Chamberlain, personal communication, 2022). First, µDys5 has five spectrin-like repeats (SRs) and two hinges as opposed to combinations of four or six SRs and three hinges in the other seven transgenes tested. Reasons to account for greater force generation using a transgene with fewer (five vs. six) SRs are not clear and were not specifically pursued in these studies. One explanation could relate to the replacement of hinge 3 in µDys5 with the entire SR23 to enhance stability and elasticity. Perhaps most importantly, µDys5 contains the nNOS binding domain found by Duan et al. to be within dystrophin SRs 16 and 17[146]. Mouse models lacking or having a mislocalized nNOS domain are at risk for functional ischemia with associated fatigue[147]. Moreover, Becker muscular dystrophy (BMD) patients with exon 42-45 deletions that include repeats 16 and 17 show mislocalization of nNOS in muscle biopsy samples and a more severe phenotype[148].
Comparing the microdystrophins that have been used in the five DMD clinical trials discussed here, only rAAV9-CK8e-μDys5 (SGT-001) contains the R16/17 SRs. Notably, the study of eight microdystrophins mentioned above[144] included a close variant (µDysH3) of an earlier transgene (µDysH2) currently used in the Sarepta and Genethon clinical trials[149]. As opposed to the five SRs and two hinges in μDys5, µDysH2 has four SRs and three hinges[149,150]. Only two of the five SRs in µDys5 are included in µDysH2. Finally, while µDys5 is driven by the CK8e cassette, the Sarepta construct employs MHCK7 and Genethon uses the synthetic Spc5-12 muscle-specific promoter[150]. Continuing the comparison, the microdystrophin in the Pfizer clinical trial was developed and tested by Xiao Xiao in both dystrophic mice[76] and dogs[81]. Called △3990, this transgene has five SRs and three hinges. A canine variation (△3849) lacking the central hinge was efficacious in mdx mice[151]. The Pfizer construct includes a muscle-specific creatine kinase promoter[152].
Ongoing DMD clinical trials are further distinguished by the AAV serotype used. The Solid and Pfizer constructs employ AAV9, whereas Genethon uses AAV-8. Sarepta utilizes AAV-rh74, which is like AAV8[149,150].
A recent clinical trial by REGENXBIO utilizes a microdystrophin that includes four SRs and three hinges plus an extended coding region of the carboxyl-terminal (CT) domain[153]. The binding of α-dystrobrevin and α-syntrophin by this CT domain may help recruit nNOS. The RGX-202 construct uses a novel AAV8 vector (NAV) Technology Platform and a muscle-specific promoter (Spc5-12)[153]. Strength measurements, histopathologic findings, immunostaining for nNOS, and MRI studies from mdx mice treated with RGX-202 suggested improvement[153].
THE WAY FORWARD
General considerations
Several expert panels offering perspectives from scientists, clinicians, regulatory bodies, funding agencies, patient advocacy groups, and industry have been convened to address the causes and mitigation of SAEs after systemic AAV-construct administration[4,5,8,33]. Given the critical role played by AAV dose in these reactions, effort has been given to engineer myotropic serotypes effective at lower doses with fewer off-target side effects compared to naturally occurring AAVs[154]. One high-throughput approach combined DNA/RNA barcoding with multiplexed next-generation sequencing to identify an AAV9 mutant called AAVMYO that specifically targets the musculature[155]. In another study, bioengineered AAV serotypes similar to AAV9 effectively targeted muscle in MTM1 knockout and mdx mice at lower doses than those necessary for standard AAV9 constructs[156]. Myotropic AAVs continue to be defined[157,158].
Importantly, the seroprevalence of antibodies against these bioengineered capsids was comparable to AAV9, suggesting they would translate to the clinic. Work done simultaneously by Byrne and his collaborators at the University of Florida showed that capsid- and genome-modified AAVrh74 vectors, originally isolated from rhesus monkeys, were more efficient than AAVrh74 controls in transducing a reporter gene in the muscle of wild-type mice[159] [Figure 7]. Providing further encouragement, titers to nonoptimized rAAVrh74 are typically negative or low in DMD patients, suggesting they may be less immunogenic[160], and this vector has been used successfully in DMD microdystrophin clinical trials[161]. To allow for a more streamlined pathway to the clinic, AAV vectors with cross-species applications have been developed[162].
Figure 7. Histogram of comparative GFP transgene RNA expression of WT vs. capsid and genomic optimized (OptX) AAVrh74 vectors injected IV into C57BL mice. Bar graphs show average of WT- and OptX-AAVrh74 △Ct across organs. Statistical significance was determined between WT and OptX (see error bars and P values). From Shoti et al., 2023[159]. Republished under Creative Commons Attribution License (CC-BY 3.0). WT: Wild-type.
Since the DMD transgene and translated dystrophin protein contribute to the immune response, mutation exclusion criteria are being critically assessed[31]. This continues a trend started for the Serepta clinical trials in which patients with DMD gene deletions in exons 8 or 9 are excluded and those with deletions in any of the exons included in the microdystrophin transgene are cautioned about an increased risk of severe immune-mediated myositis. Additional strategies have been directed at improving the efficiency of gene expression or reducing exposure to immunogenic dystrophin. For instance, studies have indicated that self-complementary single-stranded AAV vectors bypass the requirement for viral second-stranded DNA synthesis, thus lowering the required AAV dose[159,163]. Additionally, in studies completed jointly in the Stedman and Kornegay labs, the use of utrophin in mdx mice and the dystrophin null canine pointer model eliminated the T cell immune response[118] [Figure 8]. Subsequent reviews have shown potential structural and immunological advantages of utrophin, while emphasizing the need to consider amino acid sequences and domains as has been done with microdystrophins[164].
Figure 8. Schematic (A) of two German shorthaired pointer muscular dystrophy dogs (Ned and Grinch), in which the opposite cranial tibialis muscles were injected with either µ-dystrophin or µ-utrophin, showing the numbers of blood mononuclear cells reacting over time when cultured with peptides spanning each protein (B), together with the level of µ-dystrophin or µ-utrophin gene expression (C) and resulting histopathologic lesions (D). Note the marked inflammatory cell response in the AAV-µ-dystrophin treated limb (left) contrasted with the absence of inflammation in the AAV-µ-utrophin limb (right). From Song et al., 2019 (Stedman HH, corresponding author)[118].
Given the potential for mutation type to have an analogous impact on immune response in XLMTM patients receiving AAV-MTM1 constructs, consideration may also be given to using MTMR2 or other MTMR transgenes[26,122,123]. With that being said, critical surveillance for and exclusion of patients with pre-existing cholestatic liver disease will be most important.
Whether immunity is generated against AAV capsid or transgene proteins, it is essential to carefully consider immunomodulatory strategies to further reduce the risk of side effects. A variety of compounds have been used, ranging from prednisolone alone to more targeted combinatory approaches with drugs like the CD20 inhibitor rituximab[33] and complement blocker eculizumab[46].
Regarding the limitations of animal studies in predicting immune reactions, the expert panels and others have emphasized the importance of choosing species whose disease phenotype and immune functions closely resemble those of humans[46]. These findings remind us of the long-held principle that there is no perfect animal model. Indeed, in their 2020 review, Martino and Markusic concluded, “… humans will probably be the best animal model to study AAV immunity with experience gained from the use of approved AAV biologics and clinical trials[165].”
AAV-microdystrophin studies
In the ongoing microdystrophin clinical trials, SAEs have largely been manageable, although the functional effects have been mixed. Sarepta’s Elevidys (SRP-9001; delandistrogene moxeparvovec) received FDA approval in the United States in June 2023. The Embark clinical trial did not reach the primary endpoint of the North Star Ambulatory Assessment (NSAA) test, but significant benefits were observed in secondary outcomes compared to placebo. Notably, regarding the unmet needs of non-ambulatory DMD boys and young men discussed above under ethical considerations, the FDA somewhat controversially recently granted accelerated approval for the use of Elevidys in this patient group[166].
Working in collaboration with Sarepta to treat patients in Europe, Genethon has reported promising results with their microdystrophin construct, GNT0004, which employs the same transgene as Sarepta’s Elevidis, with an AAV8 serotype and a different promoter.
Solid Biosciences has moved forward with a clinical trial (NCT06138639) of their new construct, SGT-003, which incorporates a proprietary myotropic capsid, AAV-SLB10, while continuing to include the nNOS-binding R16 and R17 SRs from their original AAV9-based SGT-001. Based on data from mdx mice and NHPs, SGT-003 provides greater microdystrophin delivery to skeletal muscles and the heart.
In contrast, Pfizer recently released negative clinical data from its Phase 3 CIFFREO clinical trial (NCT04281485) of fordadistrogene movaparvovec. Treated DMD boys did not reach the primary endpoint on the NSAA test nor differ significantly compared to placebo regarding secondary outcomes. They have paused dosing due to a fatal serious adverse event in the phase 2 DAYLIGHT trial (NCT05429372).
In the RGX-202 clinical trial (NCT05693142), a three-patient cohort has been treated at a dose of 1E14 vg/kg, with a second group planned at 2E14[167,168]. Limited preliminary functional data are encouraging, and no SAEs have been reported.
Overall, these results are promising, but they highlight that the microdystrophin treatment strategy, by definition, is suboptimal. A miniaturized partially functional version of dystrophin is used and the duration and distribution of its expression remain to be well defined in patients[169-171]. A further concern was recently raised by studies showing accelerated heart involvement in a murine model with a severe cardiac phenotype, apparently due to competition between microdystrophin and utrophin at the cardiomyocyte membrane[172]. Accordingly, while efforts to optimize the microdystrophin treatment approach continue, studies of other potentially curative genetic and stem cell therapies will be pursued[169,173,174], coupled with drugs to lessen secondary effects of dystrophin deficiency such as inflammation and fibrosis[175].
AAV-MTM1 studies
The SAEs associated with AAV-MTM1 were seen in the ASPIRO clinical trial (NCT03199469) of resamirigene bilparvovec (AT132; rAAV8-Des-hMTM1) conducted initially by Audentes Therapeutics[26]. Astellas Pharma Inc. acquired Audentes in 2020 and subsequently placed a voluntary hold on dosing additional patients due to the SAEs. As part of an effort to develop alternatives for XLMTM treatment, Astellas recently licensed a new gene therapy, KT430, developed by Kate Therapeutics. KT430 utilizes a muscle-targeting myoAAV vector to deliver the MTMI gene[157].
CONCLUSION
Limitations of AAV-based therapies remind us that there is no perfect preclinical model. Gene therapy will always carry certain risks and is unique in that the effects of the drug are irreversible once delivered. The treating clinician must balance the basic medical tenet of “first, do no harm” against the inevitable progression of diseases like DMD and XLMTM. Here, careful attention must be paid to the perspective of the patients and their parents.
DECLARATIONS
Authors’ contributions
Conceived the general approach and wrote the first draft of the paper: Kornegay JN
Reviewed the paper: Stedman HH, Lawlor MW, Byrne BJ, Childers MK
Availability of data and materials
Not applicable.
Financial support and sponsorship
None.
Conflicts of interest
Kornegay JN (Texas A&M) and Byrne BJ (University of Florida) laboratories received research support from Solid Biosciences Inc. for studies by Birch et al. (2023). Kornegay JN was a paid consultant for Solid Biosciences Inc. Stedman HH is a co-founder of StrongHolt Therapeutics and an inventor on related patents in the field of gene therapy for muscle disease. Childers MK is an inventor of a patent on gene therapy for myotubular myopathy. Lawlor MW is the founder, CEO, and owner of Diverge Translational Science Laboratory. Lawlor MW is or has recently been a member of advisory boards for Solid Biosciences, Taysha Gene Therapies, and Astellas Gene Therapies (formerly Audentes Therapeutics). Lawlor MW is also a consultant for Astellas Gene Therapies (formerly Audentes Therapeutics), Encoded Therapeutics, Modis Therapeutics, Lacerta Therapeutics, Dynacure, AGADA Biosciences, Affinia Therapeutics, BioMarin, Locanabio, Vertex Pharmaceuticals, Voyager Therapeutics, and Entrada Therapeutics. Lawlor MW receives or has recently received research support from Astellas Gene Therapies, Solid Biosciences, Kate Therapeutics, Prothelia, Ecogenome, Cure Rare Disease, Rocket Pharma, Ultragenyx, Carbon Biosciences, Locanabio, Regenxbio, Vita Therapeutics, Lexeo Therapeutics, Lovelace Biomedical, Prevail Therapeutics, and Satellos.
Ethical approval and consent to participate
Not applicable.
Consent for publication
Not applicable.
Copyright
© The Author(s) 2024.
REFERENCES
1. Li X, Le Y, Zhang Z, Nian X, Liu B, Yang X. Viral vector-based gene therapy. Int J Mol Sci 2023;24:7736.
3. Mendell JR, Al-Zaidy SA, Rodino-Klapac LR, et al. Current clinical applications of in vivo gene therapy with AAVs. Mol Ther 2020;29:464-88.
4. Lek A, Atas E, Hesterlee SE, Byrne BJ, Bönnemann CG. Meeting report: 2022 muscular dystrophy association summit on 'safety and challenges in gene transfer therapy’. J Neuromuscul Dis 2023;10:327-36.
5. Lek A, Atlas E, Lin B, Hesterlee SE, et al. Meeting report: 2023 muscular dystrophy association summit on ‘safety and challenges in gene therapy of neuromuscular diseases’. J Neuromuscul Dis 2024;11:1139-60.
6. Servais L, Horton R, Saade D, Bönnemann C, Muntoni F. 261st ENMC workshop study group. 261st ENMC international workshop: management of safety issues arising following AAV gene therapy. Neuromuscul Disord 2023;33:884-96.
7. Food and Drug Administration (FDA). Cellular, tissue, and gene therapies advisory committee (CTGTAC) meeting. Available from: https://www.federalregister.gov/documents/2021/07/26/2021-15783/cellular-tissue-and-gene-therapies-advisory-committee-notice-of-meeting-establishment-of-a-public [Last accessed on 14 Oct 2024].
8. Horton RH, Saade D, Markati T, et al. A systematic review of adeno-associated virus gene therapies in neurology: the need for consistent safety monitoring of a promising treatment. J Neurol Neurosurg Psychiatry 2022;93:1276-88.
9. Chand DH, Zaidman C, Arya K, et al. Thrombotic microangiopathy following onasemnogene abeparvovec for spinal muscular atrophy: a case series. J Pediat ;231:265-8.
10. Askitopoulou H, Vgontzas AN. The relevance of the Hippocratic Oath to the ethical and moral values of contemporary medicine. Part I: the hippocratic oath from antiquity to modern times. Eur Spine J 2018;27:1481-90.
11. Askitopoulou H, Vgontzas AN. The relevance of the hippocratic oath to the ethical and moral values of contemporary medicine. Part II: interpretation of the hippocratic oath-today's perspective. Eur Spine J 2018;27:1491-500.
12. Smith CM. Origin and uses of primum non nocere - above all, do no harm! J Clin Pharmacol 2005;45:371-7.
13. Sioutis S, Reppas L, Bekos A, et al. The hippocratic oath: analysis and contemporary meaning. Orthopedics 2021;44:264-72.
15. Kane HL, Halpern MT, Squiers LB, Treiman KA, McCormack LA. Implementing and evaluating shared decision making in oncology practice. CA Cancer J Clin 2014;64:377-88.
16. Zhang M, He X, Wu J, Xie F. Differences between physician and patient preferences for cancer treatments: a systematic review. BMC Cancer 2023;23:1126.
17. Schuster ALR, Crossnohere NL, Fischer R, Furlong P, Bridges JFP. Unmet therapeutic needs of non-ambulatory patients with Duchenne muscular dystrophy: a mixed-method analysis. Ther Innov Regul Sci 2022;56:572-86.
18. Golli T, Juříková L, Sejersen T, Dixon C. The role of ataluren in the treatment of ambulatory and non-ambulatory children with nonsense mutation Duchenne muscular dystrophy - a consensus derived using a modified Delphi methodology in Eastern Europe, Greece, Israel and Sweden. BMC Neurol 2024;24:73.
19. Stedman HH, Byrne BJ. Signs of progress in gene therapy for muscular dystrophy also warrant caution. Mol Ther 2012;20:249-51.
20. Nichols TC, Whitford MH, Arruda VR, et al. Translational data from adeno-associated virus-mediated gene therapy of hemophilia B in dogs. Hum Gene Ther Clin Dev 2015;26:5-14.
21. Byrne BJ, Fuller DD, Smith BK, et al. Pompe disease gene therapy: neural manifestations require consideration of CNS directed therapy. Ann Transl Med 2019;7:290.
22. Birch SM, Lawlor MW, Conlon TJ, et al. Assessment of systemic AAV-microdystrophin gene therapy in the GRMD model of Duchenne muscular dystrophy. Sci Transl Med 2023;15:eabo1815.
23. Yue Y, Pan X, Hakim CH, et al. Safe and bodywide muscle transduction in young adult Duchenne muscular dystrophy dogs with adeno-associated virus. Hum Mol Genet 2015;15:5880-90.
24. Le Guiner C, Servais L, Montus M, et al. Long-term microdystrophin gene therapy is effective in a canine model of Duchenne muscular dystrophy. Nat Commun 2017;8:16105.
25. Mack DL, Poulard K, Goddard MA, et al. Systemic AAV8-Mediated gene therapy drives whole-body correction of myotubular myopathy in dogs. Mol Ther 2017;25:839-54.
26. Shieh PB, Kuntz NL, Dowling JJ, et al. Safety and efficacy of gene replacement therapy for X-linked myotubular myopathy (ASPIRO): a multinational, open-label, dose-escalation trial. Lancet Neurol 2023;22:1125-39.
27. Beggs AH, Böhm J, Snead E, et al. MTM1 mutation associated with X-linked myotubular myopathy in Labrador Retrievers. Natl Acad Sci U S A 2010;107:14697-702.
28. Lawlor MW, Beggs AH, Buj-Bello A, et al. Skeletal muscle pathology in X-linked myotubular myopathy: review with cross-species comparisons. J Neuropathol Exp Neurol 2016;75:102-10.
29. Lawlor MW, Schoser B, Margeta M, et al. Effects of gene replacement therapy with resamirigene bilparvovec (AT132) on skeletal muscle pathology in X-linked myotubular myopathy: results from a substudy of the ASPIRO open-label clinical trial. EBioMedicine 2024;99:104894.
30. Boehler JF, Brown KJ, Beatka M, et al. Clinical potential of microdystrophin as a surrogate endpoint. Neuromuscul Disord 2023;33:40-9.
31. Bönnemann CG, Belluscio BA, Braun S, Morris C, Singh T, Muntoni F. Dystrophin immunity after gene therapy for Duchenne's muscular dystrophy. N Engl J Med 2023;388:2294-6.
32. Zaiss AK, Cotter MJ, White LR, et al. Complement is an essential component of the immune response to adeno-associated virus vectors. J Virol 2008;82:2727-40.
33. Salabarria SM, Corti M, Coleman KE, et al. Thrombotic microangiopathy following systemic AAV administration is dependent on anti-capsid antibodies. J Clin Invest 202;134:e173510.
34. Chowdary P, Shapiro S, Makris M, et al. Phase 1-2 trial of AAVS3 gene therapy in patients with hemophilia B. N Engl J Med 2022;387:237-47.
35. Sobh M, Lagali PS, Ghiasi M, et al. Safety and efficacy of adeno-associated viral gene therapy in patients with retinal degeneration: a systematic review and meta-analysis. Transl Vis Sci Technol 2023;12:24.
36. High KA. Theodore E. Woodward Award. AAV-mediated gene transfer for hemophilia. Trans Am Clin Climatol Assoc 2003;114:337-51.
37. Nichols TC, Hough C, Agersø H, Ezban M, Lillicrap D. Canine models of inherited bleeding disorders in the development of coagulation assays, novel protein replacement and gene therapies. J Thromb Haemost 2016;14:894-905.
38. Nguyen GN, Everett JK, Kafle S, et al. A long-term study of AAV gene therapy in dogs with hemophilia a identifies clonal expansions of transduced liver cells. Nat Biotechnol 2021;39:47-55.
39. Ellinwood NM, Ausseil J, Desmaris N, et al. Safe, efficient, and reproducible gene therapy of the brain in the dog models of Sanfilippo and Hurler syndromes. Mol Ther 2011;19:251-9.
40. Bradbury AM, Gurda BL, Casal ML, et al. A review of gene therapy in canine and feline models of lysosomal storage disorders. Hum Gene Ther Clin Dev 2015;26:27-37.
41. Hordeaux J, Jeffrey BA, Jian J, et al. Efficacy and safety of a Krabbe disease gene therapy. Hum Gene Ther 2022;33:499-517.
42. Narfström K, Katz ML, Bragadottir R, et al. Functional and structural recovery of the retina after gene therapy in the RPE65 null mutation dog. Invest Ophthalmol Vis Sci 2003;44:1663-72.
43. Aguirre GD, Cideciyan AV, Dufour VL, et al. Gene therapy reforms photoreceptor structure and restores vision in NPHP5-associated Leber congenital amaurosis. Mol Ther 2021;29:2456-68.
44. Takahashi K, Kwok JC, Sato Y, Aguirre GD, Miyadera K. Extended functional rescue following AAV gene therapy in a canine model of LRIT3-congenital stationary night blindness. Vision Res 2023;209:108260.
45. Hamilton BA, Wright JF. Challenges posed by immune responses to AAV vectors: addressing root causes. Front Immunol 2021;12:675897.
46. Yang TY, Braun M, Lembke W, et al. Immunogenicity assessment of AAV-based gene therapies: An IQ consortium industry white paper. Mol Ther Methods Clin Dev 2022;26:471-94.
47. Kishimoto TK, Samulski RJ. Addressing high dose AAV toxicity - 'one and done' or 'slower and lower'? Expert Opin Biol Ther 2022;22:1067-71.
48. Mendell JR, Campbell K, Rodino-Klapac L, et al. Dystrophin immunity in Duchenne's muscular dystrophy. N Engl J Med 2010;363:1429-37.
49. Lehman AJ, Patterson WI, Davidow B, et al.
50. Jacobs AC, Hatfield KP. History of chronic toxicity and animal carcinogenicity studies for pharmaceuticals. Vet Pathol 2013;50:324-33.
51. Norman GA. Limitations of animal studies for predicting toxicity in clinical trials: Is it time to rethink our current approach? JACC Basic Transl Sci 2019;5:845-54.
52. Han JJ. FDA Modernization act 2. 0 allows for alternatives to animal testing. Artif Organs 2023;47:449-50.
54. Norman GA. Limitations of animal studies for predicting toxicity in clinical trials: part 2: potential alternatives to the use of animals in preclinical trials. JACC Basic Transl Sci 2020;5:387-97.
55. Gopinath C, Nathar TJ, Ghosh A, Hickstein DD, Nelson EJR. Contemporary animal models for human gene therapy applications. Curr Gene Ther 2015;15:531-40.
56. FDA. Guidance for industry preclinical assessment of investigational cellular and gene therapy products. Available from: https://www.federalregister.gov/documents/2013/11/25/2013-28173/guidance-for-industry-preclinical-assessment-of-investigational-cellular-and-gene-therapy-products [Last accessed on 14 Oct 2024].
57. Bailey AM, Mendicino M, Au P. An FDA perspective on preclinical development of cell-based regenerative medicine products. Nat Biotechnol 2014;32:721-3.
58. Bailey AM, Arcidiacono J, Benton KA, Taraporewala Z, Winitsky S. United States Food and Drug Administration regulation of gene and cell therapies. Adv Exp Med Biol 2015;871:1-29.
59. Brinks V, Jiskoot W, Schellekens H. Immunogenicity of therapeutic proteins: the use of animal models. Pharm Res 2011;28:2379-85.
60. Enriquez J, Mims BMD, Trasti S, Furr KL, Grisham MB. Genomic, microbial and environmental standardization in animal experimentation limiting immunological discovery. BMC Immunol 2020;21:50.
62. Kiani AK, Pheby D, Henehan G, et al. Ethical considerations regarding animal experimentation. J Prev Med Hyg 2022;63:E255-66.
63. Ziegler A, Gonzalez L, Blikslager A. Large animal models: the key to translational discovery in digestive disease research. Cell Mol Gastroenterol Hepatol 2016;2:716-24.
64. Oh JH, Cho JY. Comparative oncology: overcoming human cancer through companion animal studies. Exp Mol Med 2023;55:725-34.
65. Steenbeek FG, Hytönen MK, Leegwater PA, Lohi H. The canine era: the rise of a biomedical model. Anim Genet 2016;47:519-27.
66. Story BD, Miller ME, Bradbury AM, et al. Canine models of inherited musculoskeletal and neurodegenerative diseases. Front Vet Sci 2020;7:80.
67. Kornegay JN, Bogan JR, Bogan DJ, et al. Canine models of Duchenne muscular dystrophy and their use in therapeutic strategies. Mamm Genome 2012;23:85-108.
68. Barthélémy I, Hitte C, Tiret L. The dog model in the spotlight: legacy of a trustful cooperation. J Neuromuscul Dis 2019;6:421-51.
69. Doshi BS, Samelson-Jones BJ, Nichols TC, et al. AAV gene therapy in companion dogs with severe hemophilia: real-world long-term data on immunogenicity, efficacy, and quality of life. Mol Ther Methods Clin Dev 2024;32:101205.
70. Gareau A, Sekiguchi T, Warry E, et al. Allogeneic peripheral blood haematopoietic stem cell transplantation for the treatment of dogs with high-grade B-cell lymphoma. Vet Comp Oncol 2022;20:862-70.
71. Shin JH, Yue Y, Smith B, Duan D. Humoral immunity to AAV-6, 8, and 9 in normal and dystrophic dogs. Hum Gene Ther 2012;23:287-94.
72. Calcedo R, Franco J, Qin Q, et al. Preexisting neutralizing antibodies to adeno-associated virus capsids in large animals other than monkeys may confound in vivo gene therapy studies. Hum Gene Ther Methods 2015;26:103-5.
73. Goggs R, Behling-Kelly E. C1 inhibitor in canine intravascular hemolysis (C1INCH): study protocol for a randomized controlled trial. BMC Vet Res 2019;15:475.
74. Szebeni J, Alving CR, Rosivall L, et al. Animal models of complement-mediated hypersensitivity reactions to liposomes and other lipid-based nanoparticles. J Liposome Res 2007;17:107-17.
75. Kornegay JN, Peterson JM, Bogan DJ, et al. NBD delivery improves the disease phenotype of the golden retriever model of Duchenne muscular dystrophy. Skelet Muscle 2014;4:18.
76. Wang B, Li J, Xiao X. Adeno-associated virus vector carrying human minidystrophin genes effectively ameliorates muscular dystrophy in mdx mouse model. Proc Natl Acad Sci U S A 2000;97:13714-9.
77. Harper SQ, Hauser MA, DelloRusso C, et al. Modular flexibility of dystrophin: implications for gene therapy of Duchenne muscular dystrophy. Nat Med 2002;8:253-61.
78. Wang Z, Tapscott SJ, Chamberlain JS, Storb R. Immunity and AAV-mediated gene therapy for muscular dystrophies in large animal models and human trials. Front Microbiol 2011;2:201.
79. Wang Z, Allen JM, Riddell SR, et al. Immunity to adeno-associated virus-mediated gene transfer in a random-bred canine model of Duchenne muscular dystrophy. Hum Gene Ther 2007;18:18-26.
80. Yuasa K, Yoshimura M, Urasawa N, et al. Injection of a recombinant AAV serotype 2 into canine skeletal muscles evokes strong immune responses against transgene products. Gene Ther 2007;14:1249-60.
81. Kornegay JN, Li J, Bogan JR, et al. Widespread muscle expression of an AAV9 human mini-dystrophin vector after intravenous injection in neonatal dystrophin-deficient dogs. Mol Ther 2010;18:1501-8.
82. Li J, Qiao C, Bogan J, et al. Efficient long-term bodywide expression of an AAV9-minidystrophin in the muscle and heart of young adult GRMD dogs after intravascular injection without immune suppression. Available from: https://www.cell.com/molecular-therapy-family/molecular-therapy/pdf/S1525-0016(16)36624-2.pdf [Last accessed on 14 Oct 2024].
83. Wang Z, Kuhr CS, Allen JM, et al. Sustained AAV-mediated dystrophin expression in a canine model of Duchenne muscular dystrophy with a brief course of immunosuppression. Mol Ther 2007;15:1160-6.
84. Koo T, Okada T, Athanasopoulos T, et al. Long-term functional adeno-associated virus-microdystrophin expression in the dystrophic CXMDj dog. J Gene Med 2011;13:497-506.
85. Bowles DE, McPhee SW, Li C, et al. Phase 1 gene therapy for Duchenne muscular dystrophy using a translational optimized AAV vector. Mol Ther 2012;20:443-55.
86. Ketonis C, Ilyas AM, Liss F. Pain management strategies in hand surgery. Orthop Clin North Am 2015;46:399-408.
87. Hagstrom JE, Hegge J, Zhang G, et al. A facile nonviral method for delivering genes and siRNAs to skeletal muscle of mammalian limbs. Mol Ther 2004;10:386-98.
88. Arruda VR, Stedman HH, Haurigot V, et al. Peripheral transvenular delivery of adeno-associated viral vectors to skeletal muscle as a novel therapy for hemophilia B. Blood 2010;115:4678-88.
89. Ohshima S, Shin JH, Yuasa K, et al. Transduction efficiency and immune response associated with the administration of AAV8 vector into dog skeletal muscle. Mol Ther 2009;17:73-80.
90. Fan Z, Kocis K, Valley R, Howard JF Jr, et al. High-pressure transvenous perfusion of the upper extremity in human muscular dystrophy: a safety study with 0. 9% saline. Hum Gene Ther 2015;26:614-21.
91. Wang B, Li J, Fu FH, Xiao X. Systemic human minidystrophin gene transfer improves functions and life span of dystrophin and dystrophin/utrophin- deficient mice. J Orthop Res 2009;27:421-26.
92. Gregorevic P, Blankinship MJ, Allen JM, Chamberlain JS. Systemic microdystrophin gene delivery improves skeletal muscle structure and function in old dystrophic mdx mice. Mol Ther 2008;16:657-64.
93. Chen Y, Zheng Y, Kang Y, et al. Functional disruption of the dystrophin gene in rhesus monkey using CRISPR/Cas9. Hum Mol Genet 2015;24:3764-74.
94. Lim KRQ, Nguyen Q, Dzierlega K, Huang Y, Yokota T. CRISPR-generated animal models of Duchenne muscular dystrophy. Genes (Basel) 2020;11:342.
95. Ren S, Fu X, Guo W, et al. Profound cellular defects attribute to muscular pathogenesis in the rhesus monkey model of Duchenne muscular dystrophy. Cell 2024:S0092-8674(24)00970.
96. Rodino-Klapac LR, Janssen PM, Montgomery CL, et al. A translational approach for limb vascular delivery of the micro-dystrophin gene without high volume or high pressure for treatment of Duchenne muscular dystrophy. J Transl Med 2007;5:45.
97. Potter RA, Peterson EL, Griffin D, et al. Use of plasmapheresis to lower anti-AAV antibodies in nonhuman primates with pre-existing immunity to AAVrh74. Mol Ther Methods Clin Dev 2024;32:101195.
98. Hinderer C, Katz N, Buza EL, et al. Severe toxicity in nonhuman primates and piglets following high-dose intravenous administration of an adeno-associated virus vector expressing human SMN. Hum Gene Ther 2018;29:285-98.
99. Hordeaux J, Lamontagne RJ, Song C, et al. High-dose systemic adeno-associated virus vector administration causes liver and sinusoidal endothelial cell injury. Mol Ther 2024;32:952-68.
100. Childers MK, Joubert R, Poulard K, et al. Gene therapy prolongs survival and restores function in murine and canine models of myotubular myopathy. Sci Transl Med 2014;6:220ra10.
101. Graves SS, Storb R. Developments and translational relevance for the canine haematopoietic cell transplantation preclinical model. Vet Comp Oncol 2020;18:471-83.
102. Lupu M, Storb R. Five decades of progress in haematopoietic cell transplantation based on the preclinical canine model. Vet Comp Oncol 2007;5:14-30.
103. Graves SS, Storb R. Evolution of haematopoietic cell transplantation for canine blood disorders and a platform for solid organ transplantation. Vet Med Sci 2021;7:2156-71.
104. Gussoni E, Soneoka Y, Strickland CD, et al. Dystrophin expression in the mdx mouse restored by stem cell transplantation. Nature 1999;401:390-4.
105. Gussoni E, Bennett RR, Muskiewicz KR, et al. Long-term persistence of donor nuclei in a Duchenne muscular dystrophy patient receiving bone marrow transplantation. J Clin Invest 2002;110:807-14.
106. Dell’Agnola C, Wang Z, Storb R, Tapscott SJ, Kuhr CS, Hauschka SD, et al. Hematopoietic stem cell transplantation does not restore dystrophin expression in Duchenne muscular dystrophy dogs. Blood 2004;104:4311-8.
107. Partridge TA, Morgan JE, Coulton GR, Hoffman EP, Kunkel LM. Conversion of mdx myofibres from dystrophin-negative to -positive by injection of normal myoblasts. Nature 1989;337:176-9.
108. Tremblay JP, Malouin F, Roy R, et al. Results of a triple blind clinical study of myoblast transplantations without immunosuppressive treatment in young boys with Duchenne muscular dystrophy. Cell Transplant 1993;2:99-112.
109. Miller RG, Sharma KR, Pavlath GK, et al. Myoblast transplantation in Duchenne muscular dystrophy: the San Francisco study. Muscle Nerve 1997;20:469-78.
110. Prattis SM, Horton SB, Van Camp SD, Kornegay JN. Immunohistochemical detection of neural cell adhesion molecule and laminin in X-linked dystrophic dogs and mdx mice. J Comp Pathol 1994;110:253-66.
111. Kornegay JN, Prattis SM, Bogan DJ, et al. Results of myoblast transplantation in a canine model of muscle injury. In: Kakulas BA, Mc HJ, Roses AD, editors. Duchenne muscular dystrophy: animal models and genetic manipulation. San Diego: Raven; 1992. p. 203-12. Available from: https://www.cambridge.org/core/journals/canadian-journal-of-neurological-sciences/article/duchenne-muscular-dystrophy-animal-models-and-genetic-manipulation-1992-edited-by-kakulasba-howellj-and-rosesad-published-by-raven-press-308-pages-108-cdn-approx/DB5C25B79AE3ECFAD1A8C9136074E489 [Last accessed on 14 Oct 2024].
113. Smythe GM, Hodgetts SI, Grounds MD. Problems and solutions in myoblast transfer therapy. J Cell Mol Med 2001;5:33-47.
114. Skuk D, Paradis M, Goulet M, Tremblay JP. Ischemic central necrosis in pockets of transplanted myoblasts in nonhuman primates: implications for cell-transplantation strategies. Transplantation 2007;84:1307-15.
115. Sharp NJ, Kornegay JN, Van Camp SD, et al. An error in dystrophin mRNA processing in golden retriever muscular dystrophy, an animal homologue of Duchenne muscular dystrophy. Genomics 1992;13:115-21.
116. Schatzberg SJ, Anderson LV, Wilton SD, et al. Alternative dystrophin gene transcripts in golden retriever muscular dystrophy. Muscle Nerve 1998;21:991-8.
117. VanBelzen DJ, Malik AS, Henthorn PS, Kornegay JN, Stedman HH. Mechanism of deletion removing all dystrophin exons in a canine model for DMD implicates concerted evolution of X chromosome pseudogenes. Mol Ther Methods Clin Dev 2016;4:62-71.
118. Song Y, Morales L, Malik AS, et al. Non-immunogenic utrophin gene therapy for the treatment of muscular dystrophy animal models. Nat Med 2019;25:1505-11.
119. Laporte J, Biancalana V, Tanner SM, et al. MTM1 mutations in X-linked myotubular myopathy. Hum Mutat 2000;15:393-409.
120. Kušíková K, Šoltýsová A, Ficek A, et al. Prognostic value of genotype-phenotype correlations in X-Linked myotubular myopathy and the use of the Face2Gene application as an effective non-invasive diagnostic tool. Genes (Basel) 2023;14:2174.
121. Dowling JJ, Lawlor MW, Das S. X-Linked myotubular myopathy. In: Adam MP, Feldman J, Mirzaa GM, et al, editors. GeneReviews. Seattle 2002;25:1993-2024.
122. Raess MA, Cowling BS, Bertazzi DL, et al. Expression of the neuropathy-associated MTMR2 gene rescues MTM1-associated myopathy. Hum Mol Genet 2017;26:3736-48.
123. Danièle N, Moal C, Julien L, et al. Intravenous administration of a MTMR2-encoding AAV vector ameliorates the phenotype of myotubular myopathy in mice. J Neuropathol Exp Neurol 2018;77:282-95.
124. National Research Council. Models for biomedical research: a new perspective. Washington DC: The National Academy Press; 1985. Available from: https://nap.nationalacademies.org/catalog/19304/models-for-biomedical-research-a-new-perspective [Last accessed on 14 Oct 2024].
125. Kornegay JN, MK Childers. Canine inherited dystrophinopathies and centronuclear myopathies. In: Childers MK, editor. Regenerative medicine for degenerative muscle diseases. New York: Humana Press; 2016. p. 309-29.
126. Patronek GJ, Waters DJ, Glickman LT. Comparative longevity of pet dogs and humans: implications for gerontology research. J Gerontol A Biol Sci Med Sci 1997;52:B171-8.
127. Kornegay JN. The golden retriever model of Duchenne muscular dystrophy. Skelet Muscle 2017;7:9.
128. Tulangekar A, Sztal TE. Inflammation in duchenne muscular dystrophy-exploring the role of neutrophils in muscle damage and regeneration. Biomedicines 2021;9:1366.
129. Tripodi L, Villa C, Molinaro D, Torrente Y, Farini A. The immune system in Duchenne muscular dystrophy pathogenesis. Biomedicines 2021;9:1447.
130. Valentine BA, Cooper BJ, Cummings JF, de Lahunta A. Canine X-linked muscular dystrophy: morphologic lesions. J Neurol Sci 1990;97:1-23.
131. Kakulas BA, Adams RA. Diseases of muscle: pathological foundations of clinical Myology. 4th ed. Philadelphia: Harper & Row Publishers; 1985. p. 389-402. Available from: https://cir.nii.ac.jp/crid/1130000796164051200 [Last accessed on 14 Oct 2024].
132. Fan Z, Wang J, Ahn M, Shiloh-Malawsky Y, et al. Characteristics of magnetic resonance imaging biomarkers in a natural history study of golden retriever muscular dystrophy. Neuromuscul Disord 2014;24:178-91.
133. Kim HK, Merrow AC, Shiraj S, et al. Analysis of fatty infiltration and inflammation of the pelvic and thigh muscles in boys with Duchenne muscular dystrophy (DMD): grading of disease involvement on MR imaging and correlation with clinical assessments. Pediatr Radiol 2013;43:1327-35.
134. Shinohara I, Kataoka T, Mifune Y, et al. Influence of adiponectin and inflammatory cytokines in fatty degenerative atrophic muscle. Sci Rep 2022;12:1557.
135. Clemente-Suárez VJ, Redondo-Flórez L, Beltrán-Velasco AI, et al. The role of adipokines in health and disease. Biomedicines 2023;11:1290.
136. Snead EC, Taylor SM, van der Kooij M, et al. Clinical phenotype of X-linked myotubular myopathy in Labrador Retriever puppies. J Vet Intern Med 2015;29:254-60.
137. Jungbluth H, Wallgren-Pettersson C, Laporte J. Centronuclear (myotubular) myopathy. Orphanet J Rare Dis 200;3:26.
138. D'Amico A, Longo A, Fattori F, et al. Hepatobiliary disease in XLMTM: a common comorbidity with potential impact on treatment strategies. Orphanet J Rare Dis 2021;16:425.
139. Karolczak S, Deshwar AR, Aristegui E, et al. Loss of Mtm1 causes cholestatic liver disease in a model of X-linked myotubular myopathy. J Clin Invest 2023;133:e166275.
140. Molera C, Sarishvili T, Nascimento A, et al. Intrahepatic cholestasis is a clinically significant feature associated with natural history of X-Linked myotubular myopathy (XLMTM): a case series and biopsy report. J Neuromuscul Dis 2022;9:73-82.
141. Kodippili K, Hakim CH, Burke MJ, et al. SERCA2a overexpression improves muscle function in a canine Duchenne muscular dystrophy model. Mol Ther Methods Clin Dev 2024;32:101268.
142. Bradbury AM, Bagel J, Swain G, et al. Combination HSCT and intravenous AAV-mediated gene therapy in a canine model proves pivotal for translation of Krabbe disease therapy. Mol Ther 2024;32:44-58.
143. Franco-Martínez L, Villar M, Tvarijonaviciute A, et al. Serum proteome of dogs at subclinical and clinical onset of canine leishmaniosis. Transbound Emerg Dis 2020;67:318-27.
144. Ramos JN, Hollinger K, Bengtsson NE, Allen JM, Hauschka SD, Chamberlain JS. Development of novel micro-dystrophins with enhanced functionality. Mol Ther 2019;27:623-35.
145. Salva MZ, Himeda CL, Tai PW, et al. Design of tissue-specific regulatory cassettes for high-level rAAV-mediated expression in skeletal and cardiac muscle. Mol Ther 2007;15:320-9.
147. Kobayashi YM, Rader EP, Crawford RW, et al. Sarcolemma-localized nNOS is required to maintain activity after mild exercise. Nature 2008;456:511-5.
148. Gentil C, Leturcq F, Ben Yaou R, et al. Variable phenotype of del45-55 Becker patients correlated with nNOSμ mislocalization and RYR1 hypernitrosylation. Hum Mol Genet 2012;21:3449-60.
149. Duan DD. Systemic AAV micro-dystrophin gene therapy for Duchenne muscular dystrophy. Mol Ther 2018;26:2337-56.
150. Elangkovan N, Dickson G. Gene therapy for Duchenne muscular dystrophy. J Neuromuscul Dis 2021;8:S303-16.
151. Wang B, Li J, Qiao C, et al. A canine minidystrophin is functional and therapeutic in mdx mice. Gene Ther 2008;15:1099-106.
152. Wang B, Li J, Fu FH, et al. Construction and analysis of compact muscle-specific promoters for AAV vectors. Gene Ther 2008;15:1489-99.
153. Kim S, Buss C, Qiao C, et al. A novel AAV8-based gene therapy for Duchenne muscular dystrophy: preclinical studies in the mdx mouse. Neuromuscul Disord 2021;31:S76.
154. Srivastava A. Rationale and strategies for the development of safe and effective optimized AAV vectors for human gene therapy. Mol Ther Nucleic Acids 2023;32:949-59.
155. Weinmann J, Weis S, Sippel J, et al. Identification of a myotropic AAV by massively parallel in vivo evaluation of barcoded capsid variants. Nat Commun 2020;11:5432.
156. El Andari J, Renaud-Gabardos E, Tulalamba W, et al. Semirational bioengineering of AAV vectors with increased potency and specificity for systemic gene therapy of muscle disorders. Sci Adv 2022;8:eabn4704.
157. Tabebordbar M, Lagerborg KA, Stanton A, et al. Directed evolution of a family of AAV capsid variants enabling potent muscle-directed gene delivery across species. Cell 2021;184:4919-38.
158. Ji J, Lefebvre E, Laporte J. Comparative in vivo characterization of newly discovered myotropic adeno-associated vectors. Skelet Muscle 2024;14:9.
159. Shoti J, Qing K, Keeler GD, Duan D, Byrne BJ, Srivastava A. Development of capsid- and genome-modified optimized AAVrh74 vectors for muscle gene therapy. Mol Ther Methods Clin Dev 2023;31:101147.
160. Goedeker NL, Dharia SD, Griffin DA, et al. Evaluation of rAAVrh74 gene therapy vector seroprevalence by measurement of total binding antibodies in patients with Duchenne muscular dystrophy. Ther Adv Neurol Disord 2023;16:17562864221149781.
161. Mendell JR, Proud C, Zaidman CM, et al. Practical considerations for delandistrogene moxeparvovec gene therapy in patients with Duchenne muscular dystrophy. Pediatr Neurol 2024;153:11-18.
162. Gonzalez TJ, Simon KE, Blondel LO, et al. Cross-species evolution of a highly potent AAV variant for therapeutic gene transfer and genome editing. Nat Commun 2022;13:5947.
163. Lu Y, Ling C, Shoti J, et al. Enhanced transgene expression from single-stranded AAV vectors in human cells in vitro and in murine hepatocytes in vivo. Mol Ther Nucleic Acids 2024;35:102196.
164. Szwec S, Kapłucha Z, Chamberlain JS, Konieczny P. Dystrophin- and utrophin-based therapeutic approaches for treatment of Duchenne muscular dystrophy: a comparative review. BioDrugs 2024;38:95-119.
165. Martino AT, Markusic DM. Immune response mechanisms against AAV vectors in animal models. Mol Ther Methods Clin Dev 2019;17:198-208.
166. Bhattacharyya M, Miller LE, Miller AL, Bhattacharyya R. The FDA approval of delandistrogene moxeparvovec-rokl for Duchenne muscular dystrophy: a critical examination of the evidence and regulatory process. Expert Opin Biol Ther 2024;20:1-3.
167. REGENXBIO. REGENXBIO presents interim clinical data from Phase I/II AFFINITY DUCHENNE™ Trial of RGX-202 at 28th Annual International Congress of the World Muscle Society. Available from: https://www.prnewswire.com/news-releases/regenxbio-presents-interim-clinical-data-from-phase-iii-affinity-duchenne-trial-of-rgx-202-at-28th-annual-international-congress-of-the-world-muscle-society-301946289.html [Last accessed on 14 Oct 2024].
168. Veerapandiyan A, Rao V, Dastgir J, et al. RGX-202, an investigational gene therapy for the treatment of Duchenne muscular dystrophy: Interim clinical data (S21. 005). Neurology 2024;102:1.
169. Liang L, Sulaiman N, Yazid MD. A decade of progress in gene targeted therapeutic strategies in Duchenne muscular dystrophy: a systematic review. Front Bioeng Biotechnol 2022;10:833833.
170. Manini A, Abati E, Nuredini A, Corti S, Comi GP. Adeno-associated virus (AAV)-mediated gene therapy for Duchenne muscular dystrophy: the issue of transgene persistence. Front Neurol 2022;12:814174.
171. Chamberlain JS, Robb M, Braun S, et al. Microdystrophin expression as a surrogate endpoint for Duchenne muscular dystrophy clinical trials. Hum Gene Ther 2023;34:404-15.
172. Hart CC, Lee YI, Xie J, et al. Potential limitations of microdystrophin gene therapy for Duchenne muscular dystrophy. JCI Insight 2024;9:e165869.
173. Sun C, Shen L, Zhang Z, Xie X. Therapeutic strategies for Duchenne muscular dystrophy: an update. Genes (Basel) 2020;11:837.
174. D'Ambrosio ES, Mendell JR. Evolving therapeutic options for the treatment of Duchenne muscular dystrophy. Neurotherapeutics 2023;20:1669-81.
175. Rawls A, Diviak BK, Smith CI, Severson GW, Acosta SA, Wilson-Rawls J. Pharmacotherapeutic approaches to treatment of muscular dystrophies. Biomolecules 2023;13:1536.
176. Mendell JR, Shieh PB, McDonald CM, et al. Expression of SRP-9001 dystrophin and stabilization of motor function up to 2 years post-treatment with delandistrogene moxeparvovec gene therapy in individuals with Duchenne muscular dystrophy. Front Cell Dev Biol 2023;11:1167762.
177. Dreghici R, Redican S, Lawredne J, et al. IGNITE DMD phase I/II Study of SGT-001 microdystrophin gene therapy for DMD: 2-year outcomes update. MDA Clinical and Scientific Conference 2024; doi: 10.1016/j.nmd.2022.07.234.
178. Pfizer press release. Pfizer provides update on Phase 3 study of investigational gene therapy for ambulatory boys with Duchenne muscular dystrophy. Available from: https://www.pfizer.com/news/press-release/press-release-detail/pfizer-provides-update-phase-3-study-investigational-gene [Last accessed on 14 Oct 2024].
Cite This Article
How to Cite
Kornegay, J. N.; Stedman, H. H.; Lawlor, M. W.; Byrne, B. J.; Childers, M. K. C. First, do no harm: the role of preclinical animal models in predicting adverse events in gene therapy clinical trials for Duchenne muscular dystrophy and X-Linked myotubular myopathy. Rare. Dis. Orphan. Drugs. J. 2024, 3, 31. http://dx.doi.org/10.20517/rdodj.2024.28
Download Citation
Export Citation File:
Type of Import
Tips on Downloading Citation
Citation Manager File Format
Type of Import
Direct Import: When the Direct Import option is selected (the default state), a dialogue box will give you the option to Save or Open the downloaded citation data. Choosing Open will either launch your citation manager or give you a choice of applications with which to use the metadata. The Save option saves the file locally for later use.
Indirect Import: When the Indirect Import option is selected, the metadata is displayed and may be copied and pasted as needed.
About This Article
Special Issue
Copyright
Data & Comments
Data
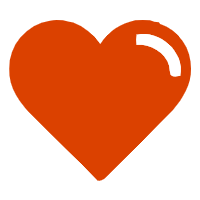
Comments
Comments must be written in English. Spam, offensive content, impersonation, and private information will not be permitted. If any comment is reported and identified as inappropriate content by OAE staff, the comment will be removed without notice. If you have any queries or need any help, please contact us at support@oaepublish.com.