Non-viral gene therapy for neuromuscular diseases including Duchenne muscular dystrophy using nanovesicles derived from human cells
Abstract
One of the biggest challenges in adeno-associated virus gene delivery for Duchenne muscular dystrophy (DMD) is that some patients cannot be treated due to pre-existing neutralizing antibodies. As an alternative, nanovesicles derived from diverse human cells have emerged as highly efficient delivery vehicles for genetic materials. This is due to their superior biocompatibility and capability to cross diverse tissue barriers. Notably, the lack of strong host immune response was witnessed in multiple preclinical studies, as well as clinical trials recently completed using human allogeneic nanovesicles. Engineering nanovesicles with tissue-specific ligands on the surface can also enhance tissue selectivity, thus reducing off-target effects. Taken together, these findings raise the possibility that this novel non-viral approach can serve as an attractive alternative to risk-prone viral-mediated gene therapy. This review discusses the recent advances in a non-viral gene therapy approach using cell-derived nanovesicles, and highlights their therapeutic potential in treating neuromuscular diseases, such as DMD, along with current challenges that need to be further addressed.
Keywords
INTRODUCTION
While causative gene defects are known for many neuromuscular diseases (NMDs), development for effective treatments remains extremely challenging. This is mainly due to the lack of safe and efficient gene delivery vehicles[1,2]. The treatment of many rare diseases (over 80% caused by monogenic mutations) is attempted by delivering wild-type copies of defective genes to patients[3]. Adeno-associated virus (AAV) has been a choice of vehicle with several FDA-approved drugs, but this approach suffers from several major challenges, such as safety concerns, cargo packaging limit, and exorbitant development costs[4-6].
Importantly, the presence of neutralizing antibodies (NAbs) and pre-existing immunity to AAV significantly diminishes the therapeutic efficacy of gene therapy and may prevent patient access caused by previous exposure or the possibility of administering repeated doses[4,5]. Furthermore, therapeutic development for NMDs is financially challenging due to small patient populations and limited markets as the majority (90%) of NMDs are classified as rare or ultrarare diseases[4,7]. These challenges have led to costs for approved therapy that can exceed a million dollars per patient[7]. Therefore, an urgent need exists to provide affordable therapeutic options for patients with NMDs.
One alternative gene delivery vehicle to AAVs is nanovesicles, nano-sized membrane-bound vesicles either released from cells to the extracellular space (e.g., extracellular vesicles, EVs) or derived from cells by other means, including cell-derived vesicles (CDVs) obtained by cell extrusion or other EV mimetics[8,9]. Derived from various human cells, nanovesicles are increasingly recognized as effective non-viral delivery systems for genetic materials due to their excellent biocompatibility and ability to penetrate different tissue barriers[8,10]. Notably, a growing number of studies have demonstrated that these nanovesicles can deliver gene payloads, including small interfering RNAs (siRNAs) and large nucleic acids like mRNAs, enabling the modulation of target genes and functional restoration in diverse tissues[11].
In this review, we will delve into the latest advancements in a non-viral gene therapy approach relying on nanovesicles derived from human cells. We will examine their potential therapeutic applications for NMDs like Duchenne muscular dystrophy (DMD) and address the ongoing challenges that need further resolution.
CHALLENGES IN CURRENT GENE THERAPY
Safety
Exposure to wild-type AAV (highly prevalent in the general population) leads to priming of the immune system against the virus, with the development of both humoral and T-cell immunity. These immune responses are augmented with increasing AAV doses, resulting in more severe outcomes: 35% of 149 AAV gene therapy clinical trials had treatment-emergent serious adverse events (TESAEs)[12]. While the side effects of gene therapy administration can be mitigated by tight monitoring and dedicated management procedures at experienced sites, safety challenges remain. For example, the regulatory authorities often put clinical trials on hold due to serious events, ranging from transient thrombocytopenia and complement activation to liver failure and patient death[13,14]. Moreover, a recent study observing sustained safety or genotoxicity over 10 years in dogs treated with AAV gene therapy found unique AAV integration events in genomic DNA and clonal expansions of transduced cells, raising long-term safety concerns about AAV-based approach[15]. In a long-term follow-up study of the first-in-human intravascular AAV gene transfer for severe hemophilia B, high-titer neutralizing antibodies with cross-reactivity to multiple serotypes persisted for up to 15 years post-infusion[16]. This contrasts with some murine, canine, and non-human primate (NHP) studies where repeat AAV administration with different serotypes was successful. The findings suggest that in humans, alternative serotypes are unlikely to evade the neutralizing antibodies developed after the initial AAV vector infusion[16].
Limited efficacy
Besides the safety issues, the limited packaging capacity of the AAV vector (~4.7 kb for conventional single-stranded AAV) is another major hurdle, which excludes many genetic disorders with large target genes[17]. Although the partial restoration of dystrophin and clinical findings upon the use of mini- or micro-dystrophin suggest that the limited packaging capacity has at least in part been overcome for DMD, this remains a challenge[18]. The extent to which AAV can transduce different cells or tissues also varies considerably. Virus capsid engineering through genetic and chemical modifications has overcome this challenge of limited tropism to many important non-hepatic tissues to a certain degree. Studies have demonstrated that mutagenesis and the insertion of high-affinity ligands, peptides, or various protein domains can modulate natural tropism and enhance targeting specificity for the central nervous system[19], various muscle tissues[20-22], and cancer cells[23]. However, these modification strategies often result in lower transduction efficiency, poor production yield, induction of anti-polymer antibody formation, and eventually, production of NAbs that can recognize modified residues[24-26]. Furthermore, the neutralizing effect of antibodies and the pre-existing immunity to AAV significantly reduce the therapeutic effects of gene therapy and preclude repeated dosing[4,5].
Cost
Finally, tremendous development and manufacturing costs for AAV vectors impose a huge barrier to patient access, particularly in rare or ultra-rare diseases with fewer patients[6,27,28]. The first AAV gene therapy, Luxturna (Spark Therapeutics), was approved in 2018 for inherited retinal dystrophy, with a cost of $425,000 per eye[29]. Since then, several AAV gene therapies have been approved, continuously setting new records for the most expensive treatments. When Zolgensma (Novartis) was introduced, it became the world's most expensive drug, costing over $2 million for a one-time treatment[30]. Later, Hemgenix (CSL Behring LLC) and, most recently, Beqvez (Pfizer) for hemophilia B were each priced at $3.5 million[31,32]. Upstaza (PTC Therapeutics) for aromatic L-amino acid decarboxylase deficiency, Roctavian (BioMarin Pharmaceutical) for hemophilia A, and Elevidys (Sarepta Therapeutics) for DMD were similarly priced at around $3 million[28,33,34].
UNIQUE ADVANTAGES OF NANOVESICLES
Nanovesicles derived from diverse human cells are emerging as highly suitable non-viral delivery vehicles for genetic materials due to their superior biocompatibility, ability to carry genetic payloads, and capability to cross diverse tissue barriers[8,10,11]. In this section, we will first discuss the three most important benefits that nanovesicles can offer to address the current challenges in gene therapy for NMDs: (1) excellent biocompatibility that allows increased safety margin and repeat dosing; (2) highly flexible drug design that enables diverse gene cargo loading as well as effective delivery to target tissues outside the liver or spleen; and (3) reduced drug cost compared to AAV-based drugs that can lower the financial barrier to develop new genetic medicine for rare disease communities, particularly NMDs.
Biocompatibility
Safety
Derived from human cells, nanovesicles are one of the most bio-friendly substances known to date, with excellent safety profiles [Figure 1]. First, nanovesicles from a wide variety of cell sources, including stem cells (e.g., mesenchymal stem cells), primary cells (e.g., fibroblasts), and immortalized cell lines (e.g., HEK293, cancer cells) from both human and animal origins, were shown to have a minimal level of toxicity in immunocompetent animals[35-37]. Following the successful demonstration of the safety and efficacy in preclinical models, nanovesicles have also been tested in humans. In recent years, the COVID-19 pandemic has accelerated the development of therapeutics and vaccines to meet urgent medical needs, and nanovesicle-based therapy is one of them. Currently, about 60 clinical trials using nanovesicles as the primary intervention are registered (www.clinicaltrials.gov), with ~30% of these trials being COVID-19-related treatments[38]. Nanovesicle-based therapeutics are also being developed to treat a wide range of other diseases, including respiratory diseases, cancer, wound healing, neurodegenerative diseases, etc., via various routes (topical, systemic, inhalation, oral, etc.). Thus far, multiple clinical studies using nanovesicles demonstrated that these novel carriers can be exceptionally well tolerated in humans [Table 1][38,39].
Figure 1. Derived from human cells, nanovesicles are highly bio-friendly substance with excellent safety profiles as compared to foreign carriers. Allogeneic nanovesicles from diverse human cell sources are well-tolerated in human patients.
Preclinical/clinical studies demonstrating the safety and feasibility of repeat dosing of nanovesicles
Species | Nanovesicles | Doses and administration routes | Key results | Ref. |
Preclinical | ||||
Wild-type C57BL/6 mice | EVs from HEK293T (+miR-199-3p) | 1st injection via i.v. and 2nd/3rd via i.p. per week for a total of 10 injections (1010 EV per dose) over 3 weeks | No visible toxicity or immune response, except a slight increase in neutrophils and a few cytokines | [36] |
Wild-type C57BL/6 mice | EVs from BJ cell line or BM-MSCs (+siRNAKras) | 108 BJ-EVs via i.p. every other day for 120 days or 109 BJ-EVs or BM-MSC-EVs every other day for 3 weeks | No adverse immune reactions (thymic depression observed from the same number of liposomes) | [37] |
mdx mice | EVs from NIH3T3 (+myostatin propeptide) | Weekly injection (20 mg/kg) via i.v. for up to 5 weeks | No overt toxicity or immunogenicity; muscle regeneration accelerated and muscle function improved by repeated treatment | [40] |
Clinical | ||||
COVID-19 patients with severe ARDS | EVs from BM-MSC (ExoFlo) | 10 mL (0.9 × 1012 EVs) or 15 mL (1.2 × 1012 EVs) per dose on days 1 and 4 | No treatment-related adverse events; 60-day mortality rate further improved after two doses | [44] |
COVID-19 patients with idiopathic or secondary facial paralysis | EVs from BM-MSC (ExoFlo) | 13 mL via i.v. and 2 mL injected into the tissue around the facial nerve per week at weeks 1, 2, and 4. | No adverse events; improved motion of affected eyelid, brow motion, and commissure over repeated treatment | [45] |
COVID-19 patients with mild to moderate symptoms | EVs from amniotic fluid (Zofin) | 1 mL via i.v. on days 0, 4, and 8 | No serious adverse events; COVID-19-related symptoms and inflammatory biomarkers improved over repeated treatment | [46] |
COVID-19 patients with severe multi-organ complications | EVs from amniotic fluid (Zofin) | 1 mL via i.v. on days 0, 4, 6, and 8 | No adverse events; ICU clinical status, respiratory symptoms, and inflammatory biomarkers improved over repeated treatment | [47] |
Chronic kidney disease patients at stage III and IV | EVs from cell-free cord-blood MSCs | 1st injection via i.v. and 2nd via intra-renal arteries a week after (100 ug/kg/dose) | No significant adverse events; inflammatory immune reaction reduced and the overall kidney function improved over repeated treatment | [48] |
Refractory ulcerative colitis or Crohn's disease patients | EVs from BM-MSC (ExoFlo) | 15 mL via i.v. in a total of 15 doses (at days 0, 2, 4, weeks 2, 6, and every 4 weeks thereafter up to week 46) | Ongoing study (approved by FDA; NCT05176366; NCT05130983) |
Repeated dosing advantage
Various preclinical and clinical studies have demonstrated the possibility of repeated administration of nanovesicles without increasing safety risks or diminishing the therapeutic effects of nanovesicle-based medicine [Table 1]. Zhu et al. conducted a comprehensive study evaluating the toxicity and immunogenicity of both WT and engineered EVs, containing miRNA cargo[36]. EVs derived from HEK293T cells were administered via intravenous and intraperitoneal routes for a total of 10 doses over 3 weeks into immune-competent C57BL/6 mice. Although a slight increase in neutrophils and a few cytokines were observed in some of the EV-treated groups compared to the PBS control group, no visible signs of abnormalities, behavioral changes, body weight changes, toxicity in organs, or appreciable immune response were observed[36]. In another study, Mendt et al. tested clinical grade EVs derived from human bone marrow-derived mesenchymal stem cells (BM-MSCs) and human foreskin fibroblasts (BJ cell line)[37]. In-depth histopathological evaluation and blood analyses revealed that the intraperitoneal administration of 108-109 EVs every other day for up to 120 days did not elicit abnormal immune reactions in mice. Additionally, the encapsulation of the siRNA payload did not affect the immune cell composition or level of cytokines. Notably, this study also showed that thymic suppression was induced by liposome treatment, but not by EVs. In a DMD mouse model, EVs harboring myostatin propeptide (EXOpro) were administered weekly for up to 5 weeks[40]. Animals treated with EXOpro displayed accelerated muscle regeneration and growth, resulting in significantly increased muscle mass and functional improvement, without eliciting toxicity or immunogenicity in mdx mice[40]. Notably, the drug effect increased with multiple doses but became less pronounced between the third and fifth injections, which appears to be due to the saturation of EXOpro binding to mature myostatin. More preclinical findings from multiple human cell sources exist to support the possible use of nanovesicle-based medicine repeatedly[41,42].
Multiple clinical studies have also reported positive results of repeated systemic nanovesicle administration for various conditions without safety concerns [Table 1][43]. BM-MSC-derived EVs (ExoFlo) have been evaluated in patients with COVID-19-associated moderate to severe acute respiratory distress syndrome (ARDS, NCT04493242, Direct Biologics, LLC). Patients were administered 10 or 15 mL of ExoFlo (0.9 × 1012 and 1.2 × 1012 EV particles per dose, respectively) on days 1 and 4, and then monitored for 60 days[44]. No treatment-related adverse events were reported from both doses, while the high-dose treatment group showed superior efficacy in median mortality, 60-day mortality rate, overall mortality, and ventilation-free days. In another pilot safety study, ExoFlo was administered to seven participants with idiopathic or secondary facial paralysis (Direct Biologics, LLC)[45]. Thirteen milliliters of ExoFlo were administered intravenously, and 2 mL was directly injected into the tissue around the facial nerve three times at weeks 1, 2, and 4. The study reported that nanovesicle treatment was effective, and no adverse events occurred. Additionally, amniotic fluid-derived EVs, administered intravenously three or four times, were well-tolerated and safe in COVID-19 patients (NCT04657406, Zofin, ZEO ScientifiX, Inc)[46,47]. Umbilical cord MSC-derived EVs were administered twice, first intravenously and then intra-arterially, which ameliorated the condition in chronic kidney disease patients without safety concerns (phase 2/3 clinical pilot study)[48]. While many of these clinical trials lack direct comparison between single and multiple dosing or statistical significance due to relatively small patient groups, it should be noted that in all cases, improved clinical outcomes were observed over repeated treatment of nanovesicle-based therapeutics. Furthermore, there are ongoing clinical trials where patients receive repeated doses of nanovesicles over a longer period, 15 doses over 46 weeks (NCT05176366 and NCT05130983, Direct Biologics, LLC). Based on current knowledge, these treatment schemes are considered safe and approved by the FDA. Comprehensive safety data on the long-term repeated administration of nanovesicles in humans are expected to be available soon, contributing to the further advancement of nanovesicle-based therapy.
To summarize, evidence indicates that nanovesicle-based therapeutics can be administered repeatedly without eliciting a strong host immune response, in stark contrast to current approaches using conventional viral vectors or lipid nanoparticles (LNPs). The favorable biocompatibility of nanovesicle-based approaches allows for flexible dosing, thus enabling alternative management of many genetic diseases, including NMDs, in which the therapeutic effect of one-time treatment may be transient.
Flexible drug design
Gene cargo loading
Among many types of muscular dystrophies, DMD is the only disease with approved gene therapy drugs. Two types of approved therapeutics reflect distinct therapeutic approaches for DMD: (1) exon-skipping drugs utilize modified antisense oligonucleotides (ASOs) to bypass mutated exons of the dystrophin gene (Amondys 45, Exondys 51, Vyondys 53, Sarepta Therapeutics; Viltepso, NS Pharma); and (2) gene replacement therapy relies on delivery of miniaturized (micro-dystrophin) functional copy packaged in AAV (Elevidys, Sarepta Therapeutics)[49]. Notably, nanovesicles have successfully been shown to deliver both short nucleic acids, such as siRNA or ASO, and large nucleic acids such as mRNAs, and therefore suit well for applications in DMD. While multiple methodologies have been tested in nanovesicles, several show relatively robust and reproducible results in various target tissues[11].
A well-established approach exists to load oligonucleotide therapeutics onto nanovesicles using lipid conjugation [Table 2][50]. By simply mixing with nanovesicles at a mild temperature (room temperature to 37 °C), oligonucleotide drugs can rapidly integrate into the membrane structure of nanovesicles driven by hydrophobic interaction between lipid moieties conjugated to oligonucleotides and membrane lipids of nanovesicles. The resulting drug/nanovesicle complex can contain thousands of drug molecules per nanovesicle[50,51]. Moreover, unloaded nucleic acids can be easily removed by subjecting the drug/nanovesicle complex to size exclusion chromatography (SEC) or other commonly used purification methods. Using this loading method, various RNA therapeutics, such as siRNA and miRNA, were shown to be delivered to target tissues and effectively modulate target gene expression in multiple indications, including Huntington’s disease[50], breast cancer[52], and ischemic brain injury[53]. The same methodology was also tested to deliver ASO therapeutics on nanovesicles (ASO-STAT6, Codiak Biosciences), which resulted in
Technologies for muscle-targeted nanovesicle drug design
Category | Technology/mechanism | Key results | Ref. |
Cargo loading | |||
siRNA/ASO | Incubation with lipid conjugated oligonucleotides | Robust loading of 2,000-3,000 copies of small nucleic acids per nanovesicle | [50-54] |
mRNA | Recruitment of cellular mRNAs using RNA binding protein motif tethered to nanovesicle membranes | Up to 7 mRNA copies per 1,000 nanovesicles; > 200-fold higher than simple overexpression of target mRNAs in the cell | [55-57] |
Complexation of nucleic acids with cationic reagents | oral/intranasal/intramuscular delivery of mRNAs produced long-lasting immunity against SARS-CoV-2 antigens | [58-60] | |
Muscle targeting | |||
Muscle-specific peptides inserted into the extracellular loop of CD63 using anchor peptide | Up to 18-fold (quadriceps) enrichment in muscle tissues; restored up to ~40% of normal dystrophin level in mdx mice | [85] | |
Muscle-specific aptamers inserted into the extracellular loop of CD63 using anchor peptide | Significantly greater muscle tissue accumulation; dystrophin restoration and functional improvements in mdx mice | [87] | |
Muscle-specific peptides fused to Lamp2b | Intramuscular injection restored both skeletal muscle wasting and cardiac function | [86] | |
Strategies to improve biodistribution | |||
Expression of CD47 on surface | Increase the blood circulation time by preventing phagocytosis by macrophages and monocytes | [113-115] | |
Decoration of surface with albumin or PEG | Enhance the blood circulation time; clinically proven strategies in multiple drugs | [118,119] | |
Pretreatment with highly biodegradable liposome | Enhance the blood circulation time by transiently occupying liver cells and saturating RES | [120] | |
Displaying tissue-specific ligands on surface | Reduction in liver accumulation and improvement of delivery efficiency in various target tissues | [67-78] | |
Taking advantage of compromised natural barriers in diseases | Enhanced permeability to various tissue lesions | [121] |
Regarding large nucleic acids, such as mRNA or plasmid DNA, at least two distinct approaches showed promising results in the preclinical studies [Table 2]. First, mRNA enrichment within nanovesicles was attempted by utilizing RNA binding protein (RBP) motifs, tethered to transmembrane proteins abundant in nanovesicle membrane such as CD63, to recruit target mRNAs expressed in the cell to nanovesicles (“active endogenous loading”)[55,56]. Recently, Zickler et al. demonstrated greatly improved mRNA loading using an optimized version of the designer Pumilio and FBF homology domain (PUFe), findings that are 2-4 times better than the previous strategy using bacteriophage MS2 coat protein (MCP) and over 200-fold higher than one can achieve from mere overexpression of the target mRNAs in cells (“passive endogenous loading”)[57]. In this study, Cre-mediated genomic editing was observed in tumor cells treated with EVs loaded with Cre mRNAs using RBP motifs, while the same amount of passively loaded control EVs showed no detectable editing. Another approach relies on charge interaction between negatively charged nucleic acid cargo and positively charged cationic lipids or polymers and then cationic complex (nucleic acids + cationic reagents) with negatively charged nanovesicle membrane (“exogenous loading”). Tsai et al. showed that EVs loaded with cationic lipid-coated mRNAs encoding immunogenic forms of the SARS-CoV-2 spike and nucleocapsid proteins induced antigen-specific CD4+ and CD8+ T-cell responses[58]. Similarly, mRNAs that encode different components of SARS-CoV-2 were successfully loaded onto various nanovesicles in studies testing mRNA vaccines for COVID-19, which resulted in the production of NAbs and adaptive immunity when administered to mice[59,60].
One of the most important advantages of nanovesicles, especially in large nucleic acid delivery, is that the loading methods discussed above are essentially agnostic of cargo sizes and can potentially be applied to large mRNAs or plasmid DNAs beyond the AAV capacity limit. While most early proof-of-concept studies tested relatively smaller reporter genes, such as EGFP (~27 kDa), Renilla luciferase (~36 kDa), or red light-emitting luciferase, Antares2 (~70 kDa), large antigens of SARS-CoV-2, such as full-length surface glycoprotein (“S protein”, ~141 kDa), were also loaded onto nanovesicles[60].
Besides the aforementioned methodologies, some exogenous loading methods, such as electroporation and sonication, were tested but often result in highly irreproducible outcomes with massive amounts of nanovesicle aggregation (observed in our own studies as well), likely due to disruption of membrane stability of nanovesicles during physical challenges[61].
Muscle-specific delivery
One of the powerful features of nanovesicles derived from various human cells is that they inherit a distinct molecular repertoire of parent cell membranes, providing unique tissue tropisms[62-66]. Moreover, such tissue-homing properties can be maximized by engineering the surface membranes of nanovesicles further. By conjugating nanobodies, aptamers, peptides, and other ligands that have a high binding affinity toward tissue-specific antigens, numerous studies have proven the engineerability of nanovesicles and consequent redistribution of customized nanovesicles to the tumor[67-74], brain[75,76], joint[77], and heart[78].
Similar approaches can be applied to steer nanovesicles toward cardiac or skeletal muscle tissues. Thus far, a handful of nanovesicle-based approaches have shown therapeutic potential for muscle diseases. However, many of these are based on relatively under-characterized activities of MSC-EVs[79-83] or delivery of protein or other gene cargo[84] without muscle-specific delivery strategy incorporated, so they will not be discussed here. There exist at least a few studies where muscle-targeted approaches result in enhanced therapeutic outcomes in DMD or other muscle diseases [Table 2][85-87].
Gao et al. used a small anchor peptide (CP05) to display on nanovesicles muscle-specific peptides (M12) and ASO drug (phosphorodiamidate morpholino oligomer, PMO) that can address the exon 23 mutation in dystrophin gene[85]. Authors first showed increased Dystrophin protein in various muscle tissues, most prominent in the quadriceps (18-fold), of dystrophin-deficient mdx mice upon systemic administration of CP05-PMO-EVs. Remarkably, the addition of muscle-specific peptide (CP05-PMO/M12-EVs) significantly enhanced the number of dystrophin-positive myofibers in most muscle tissues observed, including quadriceps, gastrocnemius, diaphragm, and abdominal muscles, and restored up to nearly 40% of normal dystrophin level (highest in gastrocnemius) from treated mdx mice. Functional rescue was also evident from force recovery in the grip strength test, without showing any liver or renal toxicity. Notably, authors described that repeated weekly injections up to 3 weeks substantially increased dystrophin expression in broad muscle tissues, enforcing the prospect of repeat dosing of nanovesicles. The same group recently reported the dystrophin restoration and functional improvements in mdx mice, like the previous report, using nanovesicles decorated with muscle-specific aptamers instead of peptides[87].
Another example described an approach using a muscle-specific peptide fused to nanovesicle marker protein, lysosomal-associated membrane protein 2b (Lamp2b), to deliver miR-26a to improve muscle wasting and cardiomyopathy that occur in chronic kidney disease (CKD)[86]. The miR-26a has been implicated in many cardiac diseases[88], and its expression was shown to be impaired in CKD mice. Intramuscular injection of nanovesicles carrying miR-26a-5p increased miR-26a expression to the normal level in the tibialis anterior (TA) muscle. Surprisingly, injection into the TA muscle also restored the miR-26a expression to the normal level in the heart. Consequently, intramuscular delivery of miR-26a not only increased the skeletal muscle cross-sectional area but also reduced cardiac fibrosis and improved cardiac function as measured by echocardiogram. Therefore, this result also supports the idea that nanovesicles can be engineered to achieve muscle-targeted delivery of gene cargo.
While these nanovesicle-based approaches remain in the preclinical stage, more advanced clinical validation of muscle-targeted engineering can be found in other drug modalities, such as antibody-conjugated oligonucleotides[89,90]. Two clinical-stage approaches centered on muscular dystrophies are particularly noteworthy: Avidity Bioscience’s approach using antibody-oligonucleotide conjugates (AOCs)[90] and Dyne Therapeutics’ Fab-conjugated ASO/PMO[89] for myotonic dystrophy type 1 (DM1), facioscapulohumeral muscular dystrophy (FSHD), and DMD. As nanovesicles offer highly flexible drug design and engineerability due to their lipid bilayer membrane structure, the majority, if not all, of currently proposed tissue-targeting strategies from other drug delivery systems[91-93] can be readily adapted to nanovesicles by decorating the external surface using chemical, genetic, and physical modifications[94].
Drug cost
The current gene therapy cost for conventional AAV-based approved drugs for DMD is prohibitively high, ranging up to $3.2M for one-time treatment per patient[28]. Such high drug cost is attributed to multiple factors: (1) massive transfection needed for AAV vector production requires high costs for materials and process development; (2) safety concerns mandate extremely stringent purification steps, for instance, to reduce empty capsids; and (3) high clinical dose requires large-scale bioreactors, imposing significant burdens on downstream processes.
In contrast, a more economical price tag is predicted for nanovesicle-based therapeutics. For instance, Piffoux et al. estimated the drug cost of engineered nanovesicle therapeutics loaded with siRNA or miRNA payloads to be in the range of €15,000-40,000[95]. This proposed cost is in a similar range as single shots for approved RNA therapeutics (annual treatment cost, however, is estimated up to $500 K)[96] and a significant cost saving compared to AAV therapy. This is likely due to the aforementioned advantages of nanovesicles that help slash manufacturing costs. While AAV often requires triple transfection[97], nanovesicles do not. As described above, gene cargo can be loaded by engineering source cells, thus allowing production through stable cell lines, or a relatively simple reaction between nanovesicles and cargo materials outside the cell. With superior safety profiles, more relaxed purification methods, such as relatively economical chromatography options, may be used (based on public information available from related conferences)[98]. Furthermore, the unique advantages of nanovesicles, such as low immunogenicity and excellent cellular uptake and tissue penetration, will directly translate to fewer materials per dose, thereby lowering production costs further.
A large body of early nanovesicle research typically relied on EVs secreted from cultured cells over extended time. Undoubtedly, the difficulty in the large-scale production of nanovesicles has prevented both academic researchers and drug developers from moving this highly promising approach into translation at full speed[11,99,100]. However, more scalable and cost-effective means exist to produce EV-like nanovesicles[9,101]. For example, BioDrone® technology (MDimune Inc.) based on cell extrusion has shown its productivity and unique therapeutic potential in multiple applications[102-106]. The extrusion of diverse cell sources yields
Figure 2. BioDrone® Platform Technology. (A) A schematic diagram shows the possible mechanism of the high productivity of CDVs compared to EVs (from Lau et al., 2022[106]). (B) Comparison of nanovesicle yield per unit cell between CDVs and EVs in diverse source cells.
To date, only one nanovesicle-based therapeutic is on the market - Bexsero, the vaccine to prevent invasive disease caused by Neisseria meningitidis serogroup B[107]. Additionally, drug costs will largely vary by several factors, such as manufacturing steps, indications, clinical dose, patient populations, etc., for which very limited information is available for comparison with other drug modalities. Therefore, more accurate estimates for drug cost can be made when more clinical programs and, finally, commercial products become available. Nonetheless, nanovesicle-based therapeutics have a high potential to bring drug costs down substantially compared to AAV vectors, enabling more affordable treatment for patients, especially those with rare and ultrarare diseases.
CHALLENGES YET TO BE ADDRESSED
Rapid clearance & hepatic distribution
One of the hurdles in achieving systemic delivery, which is required for many NMDs, is the rapid clearance of nanovesicles from circulation. When administered systemically, nanovesicles are shunted from circulation relatively fast. Using highly quantitative EVs stably expressing CD63-Nanoluciferase fusion proteins, Gupta et al. reported that 90% of the injected dose had been cleared after 5 min and was down to 0.1% 30 min post-injection, with a plasma half-life of 1.2-1.3 min[108]. EVs detected in individual organs analyzed also declined fast over time. Additional studies also estimated that EVs have a relatively short blood half-life, only a few minutes[109,110]. Interestingly, the half-life of EVs appears to be much longer in NHP, approximately 40 min, than in mice, according to Driedonks et al., who used a more sensitive reporting system based on palmitoylated EGFP-Nanoluciferase (palmGRET)[111]. The variance between NHP and mice, despite the difference in sensitivity of reporters used, warrants more careful interrogation of cross-species differences and interactions between host vs. recipient cell types.
Natural entrapment in the reticuloendothelial system (RES; e.g., liver, spleen) is largely attributed to the short circulation time of nanovesicles[112]. Multiple strategies have been tested to enhance low PK and drive non-hepatic distribution with promising preclinical outcomes [Table 2]. First, several studies showed a significant increase in half-life from nanovesicles expressing CD47 on the surface[113-115]. CD47 is a well-characterized “don’t eat me” signal that prevents phagocytosis by macrophages and monocytes. Decoration of drug carrier surface with abundant plasma protein albumin or polyethylene glycol (PEG) is a clinically approved strategy to improve the pharmacokinetic properties of various drugs[116,117]. Such approaches have also been reported to enhance the circulation time of nanovesicles[118,119]. Another interesting approach comes from the same notion that the RES system is the main consumer of drugs delivered on various nano-carriers. By pretreating animals with highly biodegradable liposome (“Nanoprimer”), which is designed to transiently occupy liver cells and saturate RES, Saunders et al. demonstrated remarkable improvement in bioavailability and delivery of the LNP-based RNA therapeutics to non-hepatic tissues[120]. It will be of great interest whether this can also help enhance the non-hepatic delivery of nanovesicles. Displaying tissue-specific ligands on nanovesicle surfaces is also a major area, which has demonstrated a reduction in liver accumulation and improvement of delivery efficiency in various target tissues as previously described[67-78]. Finally, various natural barriers are known to be compromised in many human diseases. For instance, disruption of endothelial barriers has been implicated in many diseases, including inflammation, diabetes, cardiac infarction, atherosclerosis, and infectious diseases, and leads to enhanced permeability and retention (EPR) effect in many forms of cancers[121]. Thus, such perturbation in the vascular line of defense may offer unique opportunities to increase tissue uptake of delivered drugs on nanovesicles.
Among > 40 non-COVID-19-related clinical trials, only 8 programs rely on the injection of relatively large amounts of nanovesicles via systemic administration[38]. Therefore, more extensive research will certainly be necessary to elucidate the exact fate of nanovesicles entering the system. Whether and which of these proposed strategies prove to be successful will have to be determined in further clinical trials.
Limited loading capacity vs. effective dose
Another challenge is the relatively limited payload loading capacity compared to other competing non-viral technologies, such as LNPs or antibody conjugate drugs. In most systemic approaches, approximately 1 to 10 mg of nanovesicles/kg body weight are treated in both preclinical (with a median dose of 6.75 mg/kg) and clinical studies[122,123]. Although the purity level might vary considerably by each nanovesicle preparation relying on different purification methods[124,125], 109-1010 nanovesicles/μg of total protein are generally considered to be nanovesicle products with high purity and used in many studies[126,127]. Thus, the current nanovesicle dose is equivalent to approximately 1012-1014 nanovesicles/kg, and this dose meets the current cGMP manufacturing capacity.
For small oligonucleotide therapeutics, the lipid-conjugation method can reliably encapsulate thousands of copies of siRNA or ASO on nanovesicles as described above[50,51]. When applying the current dose range above, 3,000 copies of siRNA in each nanovesicle is equivalent to 3 × 1015-3 × 1017 siRNAs/kg per dose, that is 0.066-6.6 mg/kg per dose, similar to the previous estimation[95]. Although spread over a somewhat wide dose range, this dose estimate largely overlaps with the current doses used in clinically approved RNA therapeutics as summarized in Table 3[128]. Therefore, for small oligonucleotide drugs, loading capacity does not appear to be a critical issue, although meeting clinical expectations at a lower dose range, ideally below
Comparison of currently expected dose for nanovesicle drugs with existing drugs
Gene cargo | Current dose in nanovesicles | Clinical dose of existing drugs |
siRNA | 0.066-6.6 mg/kg | Patisiran (Onpattro, Alnylam Pharmaceuticals) 0.3 mg/kg, intravenous |
Givosiran (Givlaari, Alnylam Pharmaceuticals) 2.5 mg/kg, subcutaneous | ||
Nusinersen (Spinraza, Ionis Pharmaceuticals) 12 mg/5 mL, intrathecal | ||
mRNA | 0.007-0.7 μg/kg | mRNA-3927 (NCT04159103, Phase-1/2a, Moderna) 0.3 mg/kg, intravenous |
In contrast, encapsulation of large nucleic acids, such as mRNA or plasmid DNA, presents a bigger challenge. Despite early success in proof-of-concept studies, methods to encapsulate nucleic acid payloads inside nanovesicles suffer from somewhat disappointing loading capacity. According to Zickler et al., only a few mRNA copies (2 to 7) can be loaded per 1,000 nanovesicles[57]. Again, when applying the current dose range described above, this is equivalent to 7 × 109-7 × 1011 mRNA copies/kg, or approximately
Notably, such shortcomings, especially for large nucleic acid drugs, can be compensated by other traits of nanovesicles, such as excellent cellular uptake and tissue penetration, that excel those of LNPs or AAVs. For instance, nanovesicles showed robust cellular uptake and efficient RNA cargo delivery, several orders of magnitude higher than the LNP formula used for therapeutic RNA delivery (Onpattro, Alnylam Pharmaceuticals)[129]. Additionally, nanovesicles loaded with col1a1 mRNAs induced considerably enhanced collagen engraftment and structural restoration compared to the LNP control in the dermis of photoaged mice[130]. Nawaz et al. also demonstrated that myocardial delivery of VEGF-A mRNA via nanovesicles showed a significantly higher level of VEGF-A protein production in mice compared to the same amount of mRNA delivered by LNP, implicating more efficient mRNA delivery by nanovesicles[131]. On the other hand, a series of approaches based on nanovesicle-AAV hybrids (EVs containing AAV particles naturally obtained from AAV-producer cells), pioneered by Maguire et al. demonstrated improved cellular and tissue uptake mediated by nanovesicles[132]. Previous studies have reported more than 700 times higher transduction efficiency in AAV contained within nanovesicles than AAV counterparts along with increased transduction and functional improvements in the liver, retina, hair cells, and immune cells[133-136].
Taken together, pioneering a more robust nucleic acid loading methodology, especially for large nucleic acids, is required. Since nanovesicles are derived from cells with many cellular components contained in them, it is difficult to predict the encapsulation capacity of nanovesicles solely based on particle size and compare it with other synthetic vehicles such as LNPs. However, the highly versatile structure and slightly larger size of nanovesicles (~100-150 nm) may offer room for improvement beyond the current encapsulation limit. Alternatively, the unique advantages of nanovesicles in cellular and tissue uptake can offset loading capacity deficit and possibly exert comparable therapeutic effects in a much lower dose. More evidence will be required to demonstrate such benefit.
CONCLUSION
In this review, we explored the recent progress in non-viral gene therapy approaches using nanovesicles derived from human cells. The superior safety and immunogenicity profile of nanovesicles may allow the development of a first-in-kind redosable gene therapy. Cost benefits expected for nanovesicle-based therapeutics may reduce the economic barrier to drug development for stakeholders in rare and ultra-rare NMDs. Albeit relatively early in its development stage, the unique advantages of these highly suitable nanovesicle approaches will have to be substantiated by more preclinical and clinical evidence in the coming years, especially to overcome challenges in bioavailability and large nucleic acid drug loading. If successful, nanovesicles may serve as a safe, effective, and affordable platform for numerous rare and ultrarare neuromuscular diseases, including DMD.
DECLARATIONS
Acknowledgments
We would like to thank Dr. Hui-Chong Lau and Ms. Jinhee Park at MDimune Inc. for their kind support in data analysis and literature search and Ms. Jisun Lee for graphic design in the preparation of this manuscript.
Authors’ contributions
Conceptualized and designed the review, performed literature search and data interpretation, wrote for all parts including a conclusion, and finalized the manuscript: Oh SW
Performed literature search and data interpretation, wrote for safety and repeat dosing for both preclinical and clinical studies, and reviewed the manuscript: Han J
Provided progress update on BioDrone® technology and reviewed the manuscript: Park SS
Availability of data and materials
Not applicable.
Financial support and sponsorship
None.
Conflicts of interest
Oh SW is a current employee and stockholder of Kinea Bio, Inc., and a Chief Business Advisor for MDimune Inc.; Park SS is a current employee of MDimune Inc.; Han J declared that there are no conflicts of interest.
Ethical approval and consent to participate
Not applicable.
Consent for publication
Not applicable.
Copyright
© The Author(s) 2024.
REFERENCES
1. Kempf L, Goldsmith JC, Temple R. Challenges of developing and conducting clinical trials in rare disorders. Am J Med Genet A 2018;176:773-83.
2. Tambuyzer E, Vandendriessche B, Austin CP, et al. Therapies for rare diseases: therapeutic modalities, progress and challenges ahead. Nat Rev Drug Discov 2020;19:93-111.
3. Condò I. Rare monogenic diseases: molecular pathophysiology and novel therapies. Int J Mol Sci 2022;23:6525.
4. Ledford H. Gene therapy’s comeback: how scientists are trying to make it safer. Nature 2022;606:443-4.
5. Verdera HC, Kuranda K, Mingozzi F. AAV vector immunogenicity in humans: a long journey to successful gene transfer. Mol Ther 2020;28:723-46.
6. Wong CH, Li D, Wang N, Gruber J, Lo AW, Conti RM. The estimated annual financial impact of gene therapy in the United States. Gene Ther 2023;30:761-73.
7. Yates N, Hinkel J. The economics of moonshots: value in rare disease drug development. Clin Transl Sci 2022;15:809-12.
8. Du S, Guan Y, Xie A, et al. Extracellular vesicles: a rising star for therapeutics and drug delivery. J Nanobiotechnol 2023;21:231.
9. Chen C, Wang J, Sun M, Li J, Wang HMD. Toward the next-generation phyto-nanomedicines: cell-derived nanovesicles (CDNs) for natural product delivery. Biomed Pharmacother 2022;145:112416.
10. Elliott RO, He M. Unlocking the power of exosomes for crossing biological barriers in drug delivery. Pharmaceutics 2021;13:122.
11. Cecchin R, Troyer Z, Witwer K, Morris KV. Extracellular vesicles: the next generation in gene therapy delivery. Mol Ther 2023;31:1225-30.
12. Kuzmin DA, Shutova MV, Johnston NR, et al. The clinical landscape for AAV gene therapies. Nat Rev Drug Discov 2021;20:173-4.
13. Weber T. Anti-AAV antibodies in AAV gene therapy: current challenges and possible solutions. Front Immunol 2021;12:658399.
14. Philippidis A. Novartis confirms deaths of two patients treated with gene therapy Zolgensma. Hum Gene Ther 2022;33:842-4.
15. Nguyen GN, Everett JK, Kafle S, et al. A long-term study of AAV gene therapy in dogs with hemophilia a identifies clonal expansions of transduced liver cells. Nat Biotechnol 2021;39:47-55.
16. George LA, Ragni MV, Rasko JEJ, et al. Long-term follow-up of the first in human intravascular delivery of AAV for gene transfer: AAV2-hFIX16 for severe hemophilia B. Mol Ther 2020;28:2073-82.
17. Srivastava A, Lusby EW, Berns KI. Nucleotide sequence and organization of the adeno-associated virus 2 genome. J Virol 1983;45:555-64.
18. Crudele JM, Chamberlain JS. AAV-based gene therapies for the muscular dystrophies. Hum Mol Genet 2019;28:R102-7.
19. Ghauri MS, Ou L. AAV engineering for improving tropism to the central nervous system. Biology 2023;12:186.
20. Rode L, Bär C, Groß S, et al. AAV capsid engineering identified two novel variants with improved in vivo tropism for cardiomyocytes. Mol Ther 2022;30:3601-18.
21. Weinmann J, Weis S, Sippel J, et al. Identification of a myotropic AAV by massively parallel in vivo evaluation of barcoded capsid variants. Nat Commun 2020;11:5432.
22. Tabebordbar M, Lagerborg KA, Stanton A, et al. Directed evolution of a family of AAV capsid variants enabling potent muscle-directed gene delivery across species. Cell 2021;184:4919-38.e22.
23. Hoffmann MD, Gallant JP, LeBeau AM, Schmidt D. Unlocking precision gene therapy: harnessing AAV tropism with nanobody swapping at capsid hotspots. NAR Mol Med 2024;1:ugae008.
24. Song R, Pekrun K, Khan TA, Zhang F, Paşca SP, Kay MA. Selection of rAAV vectors that cross the human blood-brain barrier and target the central nervous system using a transwell model. Mol Ther Methods Clin Dev 2022;27:73-88.
25. Arjomandnejad M, Dasgupta I, Flotte TR, Keeler AM. Immunogenicity of recombinant adeno-associated virus (AAV) vectors for gene transfer. BioDrugs 2023;37:311-29.
26. Earley J, Piletska E, Ronzitti G, Piletsky S. Evading and overcoming AAV neutralization in gene therapy. Trends Biotechnol 2023;41:836-45.
27. Domenger C, Grimm D. Next-generation AAV vectors-do not judge a virus (only) by its cover. Hum Mol Genet 2019;28:R3-14.
29. Darrow JJ. Luxturna: FDA documents reveal the value of a costly gene therapy. Drug Discov Today 2019;24:949-54.
30. Nuijten M. Pricing Zolgensma - the world’s most expensive drug. J Mark Access Health Policy 2022;10:2022353.
31. Naddaf M. Researchers welcome $3.5-million haemophilia gene therapy - but questions remain. Nature 2022;612:388-9.
32. Roy S. US FDA approves Pfizer’s gene therapy for rare bleeding disorder. 2024. Available from: https://www.reuters.com/business/healthcare-pharmaceuticals/us-fda-approves-pfizers-gene-therapy-rare-bleeding-disorder-2024-04-26/ [Last accessed on 28 Aug 2024].
33. Kansteiner F, Becker Z, Liu A, Sagonowsky E, Dunleavy K. Most expensive drugs in the US in 2023. Available from: https://www.fiercepharma.com/special-reports/priciest-drugs-2023 [Last accessed on 28 Aug 2024].
34. Liu A. BioMarin’s hemophilia gene therapy Roctavian lands FDA nod with ‘glimmers’ of enthusiasm among doctors. Available from: https://www.fiercepharma.com/pharma/biomarins-hemophilia-gene-therapy-roctavian-lands-fda-nod-glimmers-enthusiasm-among-doctors [Last accessed on 28 Aug 2024].
35. Saleh AF, Lázaro-Ibáñez E, Forsgard MAM, et al. Extracellular vesicles induce minimal hepatotoxicity and immunogenicity. Nanoscale 2019;11:6990-7001.
36. Zhu X, Badawi M, Pomeroy S, et al. Comprehensive toxicity and immunogenicity studies reveal minimal effects in mice following sustained dosing of extracellular vesicles derived from HEK293T cells. J Extracell Vesicles 2017;6:1324730.
37. Mendt M, Kamerkar S, Sugimoto H, et al. Generation and testing of clinical-grade exosomes for pancreatic cancer. JCI Insight 2018;3:99263.
38. Sanz-Ros J, Mas-Bargues C, Romero-García N, Huete-Acevedo J, Dromant M, Borrás C. Extracellular vesicles as therapeutic resources in the clinical environment. Int J Mol Sci 2023;24:2344.
39. Estes S, Konstantinov K, Young JD. Manufactured extracellular vesicles as human therapeutics: challenges, advances, and opportunities. Curr Opin Biotechnol 2022;77:102776.
40. Ran N, Gao X, Dong X, et al. Effects of exosome-mediated delivery of myostatin propeptide on functional recovery of mdx mice. Biomaterials 2020;236:119826.
41. Zhang J, Ji C, Zhang H, et al. Engineered neutrophil-derived exosome-like vesicles for targeted cancer therapy. Sci Adv 2022;8:eabj8207.
42. Reis LA, Borges FT, Simões MJ, Borges AA, Sinigaglia-Coimbra R, Schor N. Bone marrow-derived mesenchymal stem cells repaired but did not prevent gentamicin-induced acute kidney injury through paracrine effects in rats. PLoS One 2012;7:e44092.
43. Fusco C, De Rosa G, Spatocco I, et al. Extracellular vesicles as human therapeutics: a scoping review of the literature. J Extracell Vesicles 2024;13:e12433.
44. Lightner AL, Sengupta V, Qian S, et al. Bone marrow mesenchymal stem cell-derived extracellular vesicle infusion for the treatment of respiratory failure from COVID-19: a randomized, placebo-controlled dosing clinical trial. Chest 2023;164:1444-53.
45. Dreschnack PA, Belshaku I. Treatment of idiopathic facial paralysis (Bell’s palsy) and secondary facial paralysis with extracellular vesicles: a pilot safety study. BMC Neurol 2023;23:342.
46. Bellio MA, Bennett C, Arango A, et al. Proof-of-concept trial of an amniotic fluid-derived extracellular vesicle biologic for treating high risk patients with mild-to-moderate acute COVID-19 infection. Biomater Biosyst 2021;4:100031.
47. Mitrani MI, Bellio MA, Sagel A, et al. Case report: administration of amniotic fluid-derived nanoparticles in three severely Ill COVID-19 patients. Front Med 2021;8:583842.
48. Nassar W, El-Ansary M, Sabry D, et al. Umbilical cord mesenchymal stem cells derived extracellular vesicles can safely ameliorate the progression of chronic kidney diseases. Biomater Res 2016;20:21.
49. Roberts TC, Wood MJA, Davies KE. Therapeutic approaches for Duchenne muscular dystrophy. Nat Rev Drug Discov 2023;22:917-34.
50. Didiot MC, Hall LM, Coles AH, et al. Exosome-mediated delivery of hydrophobically modified siRNA for huntingtin mRNA silencing. Mol Ther 2016;24:1836-47.
51. Didiot MC, Haraszti RA, Aronin N, Khvorova A. Loading of extracellular vesicles with hydrophobically modified siRNAs. In: Patel T, editor. Extracellular RNA. New York: Springer; 2018. pp. 199-214.
52. Gong C, Tian J, Wang Z, et al. Functional exosome-mediated co-delivery of doxorubicin and hydrophobically modified microRNA 159 for triple-negative breast cancer therapy. J Nanobiotechnol 2019;17:93.
53. Zhang H, Wu J, Wu J, et al. Exosome-mediated targeted delivery of miR-210 for angiogenic therapy after cerebral ischemia in mice. J Nanobiotechnol 2019;17:29.
54. Kamerkar S, Leng C, Burenkova O, et al. Exosome-mediated genetic reprogramming of tumor-associated macrophages by exoASO-STAT6 leads to potent monotherapy antitumor activity. Sci Adv 2022;8:eabj7002.
55. Hung ME, Leonard JN. A platform for actively loading cargo RNA to elucidate limiting steps in EV-mediated delivery. J Extracell Vesicles 2016;5:31027.
56. Kojima R, Bojar D, Rizzi G, et al. Designer exosomes produced by implanted cells intracerebrally deliver therapeutic cargo for Parkinson’s disease treatment. Nat Commun 2018;9:1305.
57. Zickler AM, Liang X, Gupta D, et al. Novel endogenous engineering platform for robust loading and delivery of functional mRNA by extracellular vesicles. bioRxiv 2023.
58. Tsai SJ, Guo C, Sedgwick A, et al. Exosome-mediated mRNA delivery for SARS-CoV-2 vaccination. bioRxiv 2020.
59. Zhang Q, Wang M, Han C, et al. Intraduodenal delivery of exosome-loaded SARS-CoV-2 RBD mRNA induces a neutralizing antibody response in mice. Vaccines 2023;11:673.
60. Pomatto MAC, Gai C, Negro F, et al. Plant-derived extracellular vesicles as a delivery platform for RNA-based vaccine: feasibility study of an oral and intranasal SARS-CoV-2 vaccine. Pharmaceutics 2023;15:974.
61. Johnsen KB, Gudbergsson JM, Skov MN, et al. Evaluation of electroporation-induced adverse effects on adipose-derived stem cell exosomes. Cytotechnology 2016;68:2125-38.
62. Tang TT, Wang B, Wu M, et al. Extracellular vesicle-encapsulated IL-10 as novel nanotherapeutics against ischemic AKI. Sci Adv 2020;6:eaaz0748.
63. Skokos D, Botros HG, Demeure C, et al. Mast cell-derived exosomes induce phenotypic and functional maturation of dendritic cells and elicit specific immune responses in vivo. J Immunol 2003;170:3037-45.
64. Wahlgren J, De L. Karlson T, Brisslert M, et al. Plasma exosomes can deliver exogenous short interfering RNA to monocytes and lymphocytes. Nucleic Acids Res 2012;40:e130.
65. da C. Gonçalves F, Luk F, Korevaar SS, et al. Membrane particles generated from mesenchymal stromal cells modulate immune responses by selective targeting of pro-inflammatory monocytes. Sci Rep 2017;7:12100.
66. Choo YW, Kang M, Kim HY, et al. M1 macrophage-derived nanovesicles potentiate the anticancer efficacy of immune checkpoint inhibitors. ACS Nano 2018;12:8977-93.
67. Zhang C, Tang S, Wang M, et al. “Triple-Punch” strategy exosome-mimetic nanovesicles for triple negative breast cancer therapy. ACS Nano 2024;18:5470-82.
68. Zhang J, Song H, Dong Y, et al. Surface engineering of HEK293 cell-derived extracellular vesicles for improved pharmacokinetic profile and targeted delivery of IL-12 for the treatment of hepatocellular carcinoma. Int J Nanomedicine 2023;18:209-23.
69. Bellavia D, Raimondo S, Calabrese G, et al. Interleukin 3- receptor targeted exosomes inhibit in vitro and in vivo chronic myelogenous leukemia cell growth. Theranostics 2017;7:1333-45.
70. Gu Y, Du Y, Jiang L, et al. αvβ3 integrin-specific exosomes engineered with cyclopeptide for targeted delivery of triptolide against malignant melanoma. J Nanobiotechnol 2022;20:384.
71. Wang L, Zhou X, Zou W, et al. Exosomes containing miRNAs targeting HER2 synthesis and engineered to adhere to HER2 on tumor cells surface exhibit enhanced antitumor activity. J Nanobiotechnol 2020;18:153.
72. Huang X, Wu W, Jing D, et al. Engineered exosome as targeted lncRNA MEG3 delivery vehicles for osteosarcoma therapy. J Control Release 2022;343:107-17.
73. Han Q, Xie QR, Li F, et al. Targeted inhibition of SIRT6 via engineered exosomes impairs tumorigenesis and metastasis in prostate cancer. Theranostics 2021;11:6526-41.
74. Yang Z, Shi J, Xie J, et al. Large-scale generation of functional mRNA-encapsulating exosomes via cellular nanoporation. Nat Biomed Eng 2020;4:69-83.
75. Alvarez-Erviti L, Seow Y, Yin H, Betts C, Lakhal S, Wood MJ. Delivery of siRNA to the mouse brain by systemic injection of targeted exosomes. Nat Biotechnol 2011;29:341-5.
76. Wu T, Liu Y, Cao Y, Liu Z. Engineering macrophage exosome disguised biodegradable nanoplatform for enhanced sonodynamic therapy of glioblastoma. Adv Mater 2022;34:e2110364.
77. Liang Y, Xu X, Li X, et al. Chondrocyte-targeted MicroRNA delivery by engineered exosomes toward a cell-free osteoarthritis therapy. ACS Appl Mater Interfaces 2020;12:36938-47.
78. Mentkowski KI, Lang JK. Exosomes engineered to express a cardiomyocyte binding peptide demonstrate improved cardiac retention in vivo. Sci Rep 2019;9:10041.
79. Nakamura Y, Miyaki S, Ishitobi H, et al. Mesenchymal-stem-cell-derived exosomes accelerate skeletal muscle regeneration. FEBS Lett 2015;589:1257-65.
80. Choi JS, Yoon HI, Lee KS, et al. Exosomes from differentiating human skeletal muscle cells trigger myogenesis of stem cells and provide biochemical cues for skeletal muscle regeneration. J Control Release 2016;222:107-15.
81. Figliolini F, Ranghino A, Grange C, et al. Extracellular vesicles from adipose stem cells prevent muscle damage and inflammation in a mouse model of hind limb ischemia: role of neuregulin-1. Arterioscler Thromb Vasc Biol 2020;40:239-54.
82. Bier A, Berenstein P, Kronfeld N, et al. Placenta-derived mesenchymal stromal cells and their exosomes exert therapeutic effects in Duchenne muscular dystrophy. Biomaterials 2018;174:67-78.
83. Byun SE, Sim C, Chung Y, et al. Skeletal muscle regeneration by the exosomes of adipose tissue-derived mesenchymal stem cells. Curr Issues Mol Biol 2021;43:1473-88.
84. Chen R, Yuan W, Zheng Y, et al. Delivery of engineered extracellular vesicles with miR-29b editing system for muscle atrophy therapy. J Nanobiotechnol 2022;20:304.
85. Gao X, Ran N, Dong X, et al. Anchor peptide captures, targets, and loads exosomes of diverse origins for diagnostics and therapy. Sci Transl Med 2018;10:eaat0195.
86. Wang B, Zhang A, Wang H, et al. miR-26a limits muscle wasting and cardiac fibrosis through exosome-mediated microRNA transfer in chronic kidney disease. Theranostics 2019;9:1864-77.
87. Han G, Zhang Y, Zhong L, et al. Generalizable anchor aptamer strategy for loading nucleic acid therapeutics on exosomes. EMBO Mol Med 2024;16:1027-45.
88. Icli B, Dorbala P, Feinberg MW. An emerging role for the miR-26 family in cardiovascular disease. Trends Cardiovasc Med 2014;24:241-8.
89. Sugo T, Terada M, Oikawa T, et al. Development of antibody-siRNA conjugate targeted to cardiac and skeletal muscles. J Control Release 2016;237:1-13.
90. Malecova B, Burke RS, Cochran M, et al. Targeted tissue delivery of RNA therapeutics using antibody-oligonucleotide conjugates (AOCs). Nucleic Acids Res 2023;51:5901-10.
91. Chen J, Xu Y, Zhou M, et al. Combinatorial design of ionizable lipid nanoparticles for muscle-selective mRNA delivery with minimized off-target effects. Proc Natl Acad Sci USA 2023;120:e2309472120.
92. Kim J, Eygeris Y, Ryals RC, Jozić A, Sahay G. Strategies for non-viral vectors targeting organs beyond the liver. Nat Nanotechnol 2024;19:428-47.
93. Colapicchioni V, Millozzi F, Parolini O, Palacios D. Nanomedicine, a valuable tool for skeletal muscle disorders: challenges, promises, and limitations. Wiley Interdiscip Rev Nanomed Nanobiotechnol 2022;14:e1777.
94. Liang Y, Duan L, Lu J, Xia J. Engineering exosomes for targeted drug delivery. Theranostics 2021;11:3183-95.
95. Piffoux M, Volatron J, Silva AKA, Gazeau F. Thinking quantitatively of RNA-based information transfer via extracellular vesicles: lessons to learn for the design of RNA-loaded EVs. Pharmaceutics 2021;13:1931.
96. Sehgal I, Eells K, Hudson I. A comparison of currently approved small interfering RNA (siRNA) medications to alternative treatments by costs, indications, and medicaid coverage. Pharmacy 2024;12:58.
97. Chahal PS, Schulze E, Tran R, Montes J, Kamen AA. Production of adeno-associated virus (AAV) serotypes by transient transfection of HEK293 cell suspension cultures for gene delivery. J Virol Methods 2014;196:163-73.
98. Ahn SH, Ryu SW, Choi H, You S, Park J, Choi C. Manufacturing therapeutic exosomes: from bench to industry. Mol Cells 2022;45:284-90.
99. Herrmann IK, Wood MJA, Fuhrmann G. Extracellular vesicles as a next-generation drug delivery platform. Nat Nanotechnol 2021;16:748-59.
100. Rezaie J, Feghhi M, Etemadi T. A review on exosomes application in clinical trials: perspective, questions, and challenges. Cell Commun Signal 2022;20:145.
101. Li YJ, Wu JY, Liu J, et al. Artificial exosomes for translational nanomedicine. J Nanobiotechnol 2021;19:242.
102. Jang SC, Kim OY, Yoon CM, et al. Bioinspired exosome-mimetic nanovesicles for targeted delivery of chemotherapeutics to malignant tumors. ACS Nano 2013;7:7698-710.
103. Hong J, Kang M, Jung M, et al. T-cell-derived nanovesicles for cancer immunotherapy. Adv Mater 2021;33:e2101110.
104. Jung M, Kang M, Kim BS, et al. Nanovesicle-mediated targeted delivery of immune checkpoint blockades to potentiate therapeutic efficacy and prevent side effects. Adv Mater 2022;34:e2106516.
105. Fan J, Lee CS, Kim S, Chen C, Aghaloo T, Lee M. Generation of small RNA-modulated exosome mimetics for bone regeneration. ACS Nano 2020;14:11973-84.
106. Lau HC, Han DW, Park J, et al. GMP-compliant manufacturing of biologically active cell-derived vesicles produced by extrusion technology. J Extracell Biol 2022;1:e70.
108. Gupta D, Liang X, Pavlova S, et al. Quantification of extracellular vesicles in vitro and in vivo using sensitive bioluminescence imaging. J Extracell Vesicles 2020;9:1800222.
109. Lázaro-Ibáñez E, Faruqu FN, Saleh AF, et al. Selection of fluorescent, bioluminescent, and radioactive tracers to accurately reflect extracellular vesicle biodistribution in vivo. ACS Nano 2021;15:3212-27.
110. Kang M, Jordan V, Blenkiron C, Chamley LW. Biodistribution of extracellular vesicles following administration into animals: a systematic review. J Extracell Vesicles 2021;10:e12085.
111. Driedonks T, Jiang L, Carlson B, et al. Pharmacokinetics and biodistribution of extracellular vesicles administered intravenously and intranasally to Macaca nemestrina. J Extracell Biol 2022;1:e59.
112. Gupta D, Wiklander OPB, Wood MJA, El-Andaloussi S. Biodistribution of therapeutic extracellular vesicles. Extracell Vesicles Circ Nucleic Acids 2023;4:170-90.
113. Kamerkar S, LeBleu VS, Sugimoto H, et al. Exosomes facilitate therapeutic targeting of oncogenic KRAS in pancreatic cancer. Nature 2017;546:498-503.
114. Belhadj Z, He B, Deng H, et al. A combined “eat me/don’t eat me” strategy based on extracellular vesicles for anticancer nanomedicine. J Extracell Vesicles 2020;9:1806444.
115. Cheng L, Zhang X, Tang J, Lv Q, Liu J. Gene-engineered exosomes-thermosensitive liposomes hybrid nanovesicles by the blockade of CD47 signal for combined photothermal therapy and cancer immunotherapy. Biomaterials 2021;275:120964.
116. Sleep D, Cameron J, Evans LR. Albumin as a versatile platform for drug half-life extension. Biochim Biophys Acta 2013;1830:5526-34.
117. Mitchell MJ, Billingsley MM, Haley RM, Wechsler ME, Peppas NA, Langer R. Engineering precision nanoparticles for drug delivery. Nat Rev Drug Discov 2021;20:101-24.
118. Patras L, Ionescu AE, Munteanu C, et al. Trojan horse treatment based on PEG-coated extracellular vesicles to deliver doxorubicin to melanoma in vitro and in vivo. Cancer Biol Ther 2022;23:1-16.
119. Liang X, Niu Z, Galli V, et al. Extracellular vesicles engineered to bind albumin demonstrate extended circulation time and lymph node accumulation in mouse models. J Extracell Vesicles 2022;11:e12248.
120. Saunders NRM, Paolini MS, Fenton OS, et al. A nanoprimer to improve the systemic delivery of siRNA and mRNA. Nano Lett 2020;20:4264-9.
122. Gupta D, Zickler AM, El Andaloussi S. Dosing extracellular vesicles. Adv Drug Deliv Rev 2021;178:113961.
123. Tan F, Li X, Wang Z, Li J, Shahzad K, Zheng J. Clinical applications of stem cell-derived exosomes. Signal Transduct Target Ther 2024;9:17.
124. Patel GK, Khan MA, Zubair H, et al. Comparative analysis of exosome isolation methods using culture supernatant for optimum yield, purity and downstream applications. Sci Rep 2019;9:5335.
125. Chen J, Li P, Zhang T, et al. Review on strategies and technologies for exosome isolation and purification. Front Bioeng Biotechnol 2021;9:811971.
126. Dooley K, McConnell RE, Xu K, et al. A versatile platform for generating engineered extracellular vesicles with defined therapeutic properties. Mol Ther 2021;29:1729-43.
127. Choi H, Kim MY, Kim DH, et al. Quantitative biodistribution and pharmacokinetics study of GMP-grade exosomes labeled with (89)Zr radioisotope in mice and rats. Pharmaceutics 2022;14:1118.
128. Roberts TC, Langer R, Wood MJA. Advances in oligonucleotide drug delivery. Nat Rev Drug Discov 2020;19:673-94.
129. Murphy DE, de Jong OG, Evers MJW, Nurazizah M, Schiffelers RM, Vader P. Natural or synthetic RNA delivery: a stoichiometric comparison of extracellular vesicles and synthetic nanoparticles. Nano Lett 2021;21:1888-95.
130. You Y, Tian Y, Yang Z, et al. Intradermally delivered mRNA-encapsulating extracellular vesicles for collagen-replacement therapy. Nat Biomed Eng 2023;7:887-900.
131. Nawaz M, Heydarkhan-Hagvall S, Tangruksa B, et al. Lipid nanoparticles deliver the therapeutic VEGFA mRNA in vitro and in vivo and transform extracellular vesicles for their functional extensions. Adv Sci 2023;10:e2206187.
132. Maguire CA, Balaj L, Sivaraman S, et al. Microvesicle-associated AAV vector as a novel gene delivery system. Mol Ther 2012;20:960-71.
133. György B, Fitzpatrick Z, Crommentuijn MHW, Mu D, Maguire CA. Naturally enveloped AAV vectors for shielding neutralizing antibodies and robust gene delivery in vivo. Biomaterials 2014;35:7598-609.
134. Wassmer SJ, Carvalho LS, György B, Vandenberghe LH, Maguire CA. Exosome-associated AAV2 vector mediates robust gene delivery into the murine retina upon intravitreal injection. Sci Rep 2017;7:45329.
135. György B, Sage C, Indzhykulian AA, et al. Rescue of hearing by gene delivery to inner-ear hair cells using exosome-associated AAV. Mol Ther 2017;25:379-91.
Cite This Article
How to Cite
Oh, S. W.; Han J.; Park S. S. Non-viral gene therapy for neuromuscular diseases including Duchenne muscular dystrophy using nanovesicles derived from human cells. Rare. Dis. Orphan. Drugs. J. 2024, 3, 26. http://dx.doi.org/10.20517/rdodj.2024.16
Download Citation
Export Citation File:
Type of Import
Tips on Downloading Citation
Citation Manager File Format
Type of Import
Direct Import: When the Direct Import option is selected (the default state), a dialogue box will give you the option to Save or Open the downloaded citation data. Choosing Open will either launch your citation manager or give you a choice of applications with which to use the metadata. The Save option saves the file locally for later use.
Indirect Import: When the Indirect Import option is selected, the metadata is displayed and may be copied and pasted as needed.
About This Article
Special Issue
Copyright
Data & Comments
Data
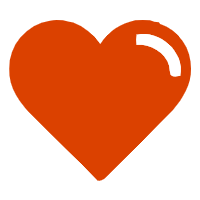
Comments
Comments must be written in English. Spam, offensive content, impersonation, and private information will not be permitted. If any comment is reported and identified as inappropriate content by OAE staff, the comment will be removed without notice. If you have any queries or need any help, please contact us at support@oaepublish.com.