Health risk assessment of humans exposed to soil and dust trace elements from e-waste sites in Bangladesh
Abstract
Elevated levels of trace elements are thought to pose a serious health risk to workers in e-waste recycling facilities. We evaluated the distribution, contamination, and human health risks of trace elements in soil and dust from e-waste exposed and non-exposed sites in Bangladesh. Thirty-one soil (20 exposed; 11 non-exposed) and 31 dust samples (21 exposed; 10 non-exposed) were collected and analyzed for Pb, Cd, Hg, As, Cr, Cu, Mn, Ni, Zn, Be, Co, Se, and V using ICP-MS. The mean concentrations of Pb, Hg, Cu, and Zn concentrations were significantly higher in soil (587, 25.57, 2,912, 894 mg/kg) and dust (439, 59, 617, 1,201 mg/kg) than in non-exposed soil (15.94, 0.07, 23.71, 121 mg/kg; all P: ≤ 0.001) and dust (15, 3.56, 19, 213 mg/kg; all P: ≤ 0.001). Children were more vulnerable than adults to non-carcinogenic risks from ingestion, while non-carcinogenic risks from dermal and inhalation exposure were negligible. Pb, Hg, and Cu from soil, as well as Pb, Hg, and Cr from dust, showed a hazard index (HI) greater than 1, indicating a risk higher than the acceptable level for children and adults at the exposed sites, while the risk to a reference child and adults was negligible. Cr and Ni contributed to cancer risk only when their concentrations reached their maximum level, exceeding the permissible limit of 1 × 10-4. This study demonstrates a significant accumulation of trace elements in e-waste recycling sites, where exposure through soil and dust poses environmental and human health risks, particularly for children. This underscores an urgent need for regulatory authorities to implement control measures and deploy awareness programs to limit trace element pollution and mitigate its impacts on the environment and human health.
Keywords
INTRODUCTION
E-waste is a fast-expanding waste stream that poses serious risks to both the environment and public health[1]. The exponential growth of the electronics industry in recent decades has led to a disproportionate amount of obsolete waste. In 2019, approximately 53.6 million metric tonnes (MMT) of e-waste were generated globally, which is projected to rise to 74.7 MMT by 2030[2]. Informal e-waste recycling processes, such as roasting, burning, acid leaching, shredding, and dumping, significantly increase the amount of hazardous elements released into the environment[3]. A wide variety of items, including computers, mobile phones, circuit boards, fluorescent lights, electric cable wires, and small household parts, are recycled at informal facilities using primitive techniques. These methods potentially release toxic chemical elements, including toxic metals such as lead, cadmium, chromium, mercury, copper, manganese, nickel, arsenic, zinc, iron, and aluminum. In addition, persistent organic pollutants (POPs), including brominated flame retardants, polybrominated diphenyls, brominated diphenyl ethers, polychlorinated biphenyls, and others, are also found in electronic components[4]. Workers handling e-waste are directly exposed through inhalation, ingestion, and dermal absorption, although the contribution of each exposure route may differ based on the substances and recycling processes involved[5].
Pollutants from e-waste are resistant to decomposition by biological processes, persistent in the environment, and can often spread to adjacent areas[6]. These hazardous chemicals produced from e-waste recycling deteriorate air quality, contaminate food, leach into groundwater, and damage ecosystems[7,8]. For example, during rainfall, trace metals in the soil can be carried away and end up in water bodies far from the recycling facility. They can also contaminate local groundwater through leaching, particularly in acidic environments[9,10]. Studies of toxic metal contamination at e-waste recycling sites have shown concentrations often exceeding national and international safety limits, with high levels detected in air, dust, soil, and plants[11]. Evaluation of soil and dust in various e-waste recycling hotspots have similarly reported elevated concentrations of toxic elements, which pose both carcinogenic and non-carcinogenic health risks from prolonged exposure[12-14].
In Bangladesh, approximately 3 MMT of e-waste is generated every year, with a growth rate of around 20% annually[15,16]. Most e-waste recycling operations occur in informal facilities, where valuable metals are quickly recovered using primitive methods without safety gear or appropriate technology[17]. Earlier evidence reported elevated concentrations of toxic metals among e-waste recycling workers in similar settings[18]. It is essential to evaluate the levels of toxic elements in environmental media where the population is directly exposed. To our knowledge, no research has compared the concentrations of various trace elements in soil and dust from e-waste exposed to non-exposed environments in Bangladesh. This study focuses on Dhaka, Bangladesh, to assess the risk and contamination of surface soils and dust by trace elements resulting from uncontrolled e-waste recycling. The study aims to measure (1) the concentrations of lead (Pb), cadmium (Cd), mercury (Hg), arsenic (As), chromium (Cr), copper (Cu), manganese (Mn), nickel (Ni), zinc (Zn), beryllium (Be), cobalt (Co), selenium (Se), and vanadium (V) in soil and dust; (2) the pollution level at e-waste-exposed sites using a geo-accumulation index (Igeo); and (3) the non-carcinogenic and carcinogenic risks in both e-waste exposed and non-exposed sites using the United States Environmental Protection Agency (US-EPA) health risk assessment model. The findings of the study will address existing knowledge gaps regarding environmental metal exposure, ascertain the most important contributors to metal contamination, and provide the foundation for environmental health standards and criteria for Bangladesh. Furthermore, the outcomes of this study can also be used to monitor environmental contamination of e-waste recycling areas and to facilitate the development of appropriate measures to reduce contamination.
MATERIALS AND METHODS
Sampling location, collection
This study included e-waste recycling sites in Dhaka and a non-exposed control site in Tangail, where no e-waste sources existed and industrial exposure was limited[19]. E-waste recycling operations typically involve manual dismantling with tools such as hammers and screwdrivers, open-air burning, acid leaching, and others without the use of protective equipment. Most recycling activities occur within business settlements alongside other operations, and residential neighborhoods are located in close proximity [Figure 1]. A trained team collected soil samples (n = 20) from different e-waste-exposed sites where activities such as combustion, dismantling, storage, and disposal were performed. Ten control samples were collected from non-exposed sites. To collect soil samples, fieldworkers cleaned a 1 m2 area with a stainless-steel spade, removing visible dust, paper, plastic, and other debris. This cleaned surface was then divided into six equal quadrangles using the hand trowel. The team collected six subsamples from the 6 quadrangles using a stainless-steel spade. In total, 100 to 150 g of soil was collected from the top surface layer of soil at a depth of 0 to 6 cm.
In addition, indoor dust samples were collected from 20 e-waste processing shops and 11 non-exposed shops. The team instructed workers to collect dust samples at the end of a workday, prior to any cleaning activities. Using precleaned paintbrushes, they swept dust from the floor, desks, and shelves and collected it in a dustpan. The samples were then mixed thoroughly with a hand trowel, and 100 to 150 g of homogenous dust was transferred into an airtight Ziplock plastic bag to protect it from ambient moisture and contaminants[19]. All sampled locations were recorded using GPS coordinates, which were then used to develop a site map using ArcGIS mapping software [Figure 1]. All samples were labeled, transported, and stored at room temperature at the icddr,b facility.
Elemental analysis
Soil and dust samples were analyzed using inductively coupled plasma mass spectrometry (ICP-MS; PerkinElmer, Model: NexION 2000; USA) following acid digestion at the Bangladesh Council of Scientific and Industrial Research (BCSIR). Samples were first dried to constant weight at 105 °C, disaggregated using a mortar and pestle, and then sieved to less than 2 mm. Following the US-EPA Method 3051, samples were acid-digested using a microwave oven digester. The detailed extraction technique for trace metals using microwave digestion is described elsewhere[20]. Around 0.3 g of soil or dust samples were placed into a tetrafluoro methoxyl (TFM) vessel placed on the balanced plate. Under a safety shield, 6 mL of 65% ultrapure nitric acid (HNO3) and 2 mL of 98% sulfuric acid (H2SO4) were added to TFM vessels in accordance with microwave digestion application note HPR-EN-36. The vessels were sealed, placed in the rotor, and microwaved for 30 min at 200 °C. After cooling the rotor to room temperature, the solution in the vessel was extracted with deionized water and transferred to a labeled volumetric flask (25 mL). The sample was then filtered and preserved for ICP-MS analysis. The nebulized sample entered (~0.90 mL/min) the core of ICP-MS system’s argon (Ar) plasma. The mass-to-charge ratio (m/z) of the trace metal ions generated in the high-temperature plasma was measured using a quadrupole mass detector. A standard calibration curve was prepared using an ICP-multi-element standard solution XIII (Merck, Germany) at ppb level.
Quality assurance and quality control
To reduce the chance of contamination during sample preparation and analytical procedures, all the glassware used in this experiment was washed and rinsed with ultrapure nitric acid and water. Prior to calibration with standards, the performance of ICPMS equipment was checked using the NexION Setup solution (PerkinElmer, USA). A five-point calibration variance, R2 > 0.9995, was attained over a dynamic range of 1.0-50 ng·mL-1. To ensure instrument stability, at least three replicates were performed, with a maximum relative standard deviation (RSD) of 5%-8%. The detection (DL) and quantification (QL) limits of the instruments were defined when the response for a certain trace element was three and ten times greater than the background noise, respectively. The recovery percentage of the elements in the samples was checked using a standard reference material SRM 1648a (for dust) from the National Institute of Standards (NIST), which indicated 92%-109.3% recovery for significant elements. As part of the quality control technique, procedure blanks, reagent blanks, SRM recoveries, and spike recoveries were carried out to reduce errors. The trace element concentrations were adjusted based on the analysis of recovery and blank samples.
Data analysis
Soil and dust trace element concentrations are presented as minimum, maximum, 50th percentile, mean, standard deviation (SD), and coefficient of variation (CV). We used the Student t-test (2-tailed) to compare normally distributed data, while the Mann-Whitney U test was used for nonnormal or skewed data for two-group comparison. Differences were considered statistically significant when P ≤ 0.05 (two-tailed). We used statistical software STATA 17 (release 21; StataCorp) and MS Excel 2021. Figures were drawn using R software (version 3.5.2 for Windows; R Foundation for Statistical Computing).
Igeo
To determine the contamination level of both dust and soil samples, we calculated the Igeo[21]. This index is useful for evaluating anthropogenic contamination, and Igeo was calculated using Equation (1):
where Cn is the total concentration of element “n” in the surface layer of both soil and dust samples, and Bn is the concentration of element “n” in the background samples, which is the non-exposed control site sample values. A factor of 1.5 is used to account for the possible variations of the background data due to lithological variations. Finally, the Igeo is grouped into seven classes[22] [Supplementary Table 1].
Health risk assessment
A health risk assessment was conducted to estimate the impacts on human health and ascertain whether these effects might result from non-carcinogenic or carcinogenic chemical exposures[23]. Human health risk models have proven effective and are widely adopted to present risk information to the decision-maker[24]. Currently, Bangladesh does not have established permissible limits for carcinogenic and non-carcinogenic risks. Therefore, in this study, we used the US-EPA model to evaluate the potential human health risks posed by trace element pollution.
Chronic daily intake (CDI, mg/kg/day) was used to estimate the extent of exposure to trace elements for both children and adults through ingestion, inhalation, and dermal pathways. Separate calculations were made for children and adults due to behavioral and physiological differences[25]. All three pathways of soil and dust intake were considered, and CDI was estimated using the standardized method and factors outlined in Table 1[26].
Trace element exposure factors
Value | |||||
Factor | Unit | Definition | Children | Adults | Ref. |
IngR | mg/day | Ingestion rate of soil | 200 | 100 | [23,27] |
EF | days/year | Exposure frequency | 350 | 350 | [23,28] |
ED | years | Exposure duration | 6 | 24 | [29,30] |
BW | kg | Average body weight | 15 | 70 | [31,32] |
AT | days | Average time (for non-carcinogens) | 365 × ED | 365 × ED | [28,33,34] |
AT | days | Average time (for carcinogens) | 365 × 70 | 365 × 70 | [33,34] |
CF | kg/mg | Conversion factor | 1 × 10-6 | 1 × 10-6 | [28,35,36] |
InhR | m3/day | Inhalation rate | 7.6 | 20 | [13,37,38] |
SA | cm2 | Surface area of the skin that contacts the dust | 2,800 | 5,700 | [13,39-41] |
AF | mg/cm2/day | Skin adherence factor | 0.2 | 0.07 | [13,30,39] |
ABF | - | Dermal adsorption factor | 0.001 | 0.001 | [41,42] |
PEF | m3/kg | Particle emission factor | 1.36 × 109 | 1.36 × 109 | [23,43] |
To calculate the CDI for each exposure pathway, the exposure frequency (EF) and surface area (SA) were obtained from country-specific data[13,28,41]. Body weight (BW) values were taken from the Bangladesh Health and Demographic Survey (BDHS), with 15 kg for children and 70 kg for adults[31,32]. Other exposure parameters were obtained from relevant literature. The following Equations (2-5) were used to determine the CDI for each of the three exposure pathways.
Risk calculation
The hazard quotient (HQ) was used to compute the non-carcinogenic health risk. The HQ for a given element was calculated by dividing the CDI values for each exposure pathway by the reference dose (RfD), as shown in Equation (6). The RfD (mg/kg/day) is an estimate of the daily exposure to the population [Table 2].
RfD (mg·kg-1·day-1) | SF (mg·kg-1·day-1) | |||||
Metals | Ingestion | Inhalation | Dermal | Ingestion | Inhalation | Dermal |
Pb | 3.50 × 10-03 | 3.52 × 10-03 | 5.25 × 10-04 | 0.0085 | 0.0420 | 0.4250 |
Cd | 1.00 × 10-03 | 1.00 × 10-03 | 1.00 × 10-05 | 0.38 | 6.30 | - |
Hg | 3.00 × 10-04 | 3.00 × 10-04 | 2.40 × 10-05 | |||
As | 3.00 × 10-04 | 1.23 × 10-04 | 3.00 × 10-04 | 1.50 | 15.10 | 1.50 |
Cr | 3.00 × 10-03 | 2.86 × 10-05 | 6.00 × 10-05 | 0.50 | 41.00 | 2.10 |
Cu | 3.70 × 10-02 | 4.02 × 10-02 | 1.20 × 10-02 | |||
Mn | 1.40 × 10-01 | 1.43 × 10-05 | 1.84 × 10-03 | |||
Ni | 2.00 × 10-02 | 2.06 × 10-02 | 5.40 × 10-03 | 0.91 | 0.84 | 4.55 |
The computed HQ values for each exposure pathway were then summed to express the non-carcinogenic risk as a hazard index (HI) [Equation (7)]. When HI is ≤ 1, the exposed individual is unlikely to experience an apparent detrimental health effect. Conversely, HI value > 1 indicates a potential adverse health effect.
Carcinogenic risks were assessed by estimating the incremental probability of an individual developing cancer over their lifetime due to exposure to carcinogens[45]. The cancer risk for each trace element was determined by multiplying the CDI values for each exposure route by the cancer slope factor (CSF) for that trace element and exposure route. According to the International Agency for Research on Cancer (IARC), Cd, Pb, As, Ni, and Cr are classified as carcinogenic elements, and CSF values are available only for these elements [Table 2][46]. Therefore, the carcinogenic risk and total carcinogenic risk (TCR) for these elements from the three exposure pathways were determined using Equations (8 and 9):
The slope factor (SF) of trace elements (with units of mg/kg/day) was used in this calculation. The USEPA’s acceptable threshold for TCR ranges from 1 × 10-6 to 1 × 10-4 for regulatory purposes, with TCR value exceeding 1 × 10-4 considered unacceptable and likely harmful to human health.
RESULTS AND DISCUSSION
Trace element concentrations in soil
Table 3 presents the elemental concentrations of soil from both exposed and non-exposed sites and compares soil concentrations with other reference values. The exposed soil sites were further categorized into combustion, dismantling, disposal, and storage sites, as shown in Supplementary Table 2. Since safe trace element levels for soil in Bangladesh have not yet been established, trace metal concentrations in soil samples were compared to other guidelines, including average shale values, WHO limits, USEPA limits, Dutch soil guidelines, and findings from e-waste studies in India, China, and Ghana [Table 3]. Pb, Cd, Hg, Cu, and Zn were the most abundant elements in exposed soil, significantly higher than their respective concentrations in the non-exposed control sites (P ≤ 0.001). The average concentrations of Pb, Cd, Hg, Cu, and Zn also exceeded the corresponding limits of WHO, USEPA, and Dutch soil guidelines, as well as the concentrations observed in studies from other settings[52,53]. Compared to non-exposed soil, the mean concentrations of Pb, Cd, Hg, Cu, and Zn were approximately 37, 50, 82, 123, and 7 times higher, respectively, in exposed soil. Additionally, the mean concentrations of Cr and Mn were 30.06 and 255 mg/kg, respectively, both exceeding the USEPA threshold values. Similarly, the mean Ni concentration surpassed the Dutch soil guideline value. The order of mean concentrations of the abundant trace elements in exposed sites was as follows: Cu > Zn > Pb > Mn > Ni > Cr > Hg > V > Co > As > Cd > Se > Be. Coefficients of variation (CV) for Pb, Cd, Hg, and Cu were 1.63, 1.35, 1.20, and 2.33, respectively, reflecting the elevated concentrations of trace elements in soil samples. These wide concentration ranges and high CV values indicate that the trace elements are strongly related to anthropogenesis[53]. This finding was somewhat similar to the concentrations in Ghanaian soils around e-waste recycling facilities[53]. The accumulation of trace metals in exposed soils likely persists due to the depletion of natural soil components, such as minerals and microbes, caused by intense e-waste processing activities, which can pose risks to both human health and the environment[54]. In our earlier analysis of workers exposed to e-waste, we found that soil Pb concentrations were associated with increased blood Pb levels, which are correlated to health risks, including impaired renal, liver and cardiovascular function[18,55,56]. This suggests an increased risk of adverse effects from metal exposure among recycling workers. It is important to note that the e-waste recycling sites are not located in a specific location in Dhaka; instead, they are typically small, mobile workshops known as “vangari shops” located in residential areas or business establishments where people regularly disassemble goods both inside and outside of the workshops[57]. As a consequence, local residents, in addition to workers, are frequently exposed to toxic elements. Even though recycling activities are localized, research has shown that hazardous substances from e-waste can spread beyond the processing sites into the surrounding ecosystems[58]. The mean values of As, Mn, Co, Se, and V were negligible due to their very low concentrations at the exposed sites. However, at one recycling site, we observed a significantly higher concentration of soil Ni (199 mg/kg), exceeding WHO and USEPA limits.
Trace element concentrations in soil (mg/kg)
Cu | Zn | Pb | Mn | Ni | Cr | Hg | V | Co | As | Cd | Se | Be | |
Exposed sites (n = 20) | |||||||||||||
Min | 35.70 | 56.63 | 15.41 | 122 | 12.16 | 12.37 | 0.83 | 8.08 | 4.49 | 1.65 | 0.10 | 0.27 | 0.12 |
Max | 29,345 | 3,178 | 4,191 | 474 | 119.27 | 72.39 | 123 | 17.73 | 10.18 | 15.94 | 19.42 | 0.93 | 0.30 |
50th percentile | 432 | 681 | 316 | 224 | 26.78 | 27.94 | 10.70 | 12.50 | 5.94 | 3.33 | 1.57 | 0.42 | 0.23 |
Average | 2,912 | 894 | 587 | 255 | 39.12 | 30.06 | 25.57 | 12.84 | 6.37 | 4.33 | 3.54 | 0.46 | 0.23 |
SD | 6,790 | 753 | 954 | 100 | 28.48 | 14.70 | 30.70 | 2.42 | 1.64 | 3.41 | 4.79 | 0.16 | 0.05 |
CV | 2.33 | 0.84 | 1.63 | 0.39 | 0.73 | 0.49 | 1.20 | 0.19 | 0.26 | 0.79 | 1.35 | 0.34 | 0.20 |
Non-exposed site (n = 11) | |||||||||||||
Min | 6.71 | 44.70 | 8.93 | 133 | 4.59 | 9.06 | 0.00 | 12.00 | 2.75 | 1.43 | 0.00 | 0.21 | 0.23 |
Max | 111 | 284 | 32.55 | 444 | 14.30 | 23.98 | 1.52 | 27.41 | 8.71 | 2.96 | 0.21 | 0.95 | 0.71 |
50th percentile | 12.65 | 87.25 | 14.88 | 205 | 8.85 | 11.96 | 0.00 | 16.24 | 5.15 | 2.14 | 0.07 | 0.52 | 0.40 |
Average | 23.71 | 121 | 15.94 | 233 | 8.99 | 13.14 | 0.31 | 17.55 | 5.65 | 2.19 | 0.07 | 0.55 | 0.44 |
SD | 29.93 | 83 | 7.85 | 93.64 | 2.53 | 4.42 | 0.47 | 4.61 | 2.16 | 0.54 | 0.06 | 0.21 | 0.16 |
CV | 1.26 | 0.69 | 0.49 | 0.40 | 0.28 | 0.34 | 1.51 | 0.26 | 0.38 | 0.25 | 0.86 | 0.39 | 0.36 |
P-value | ≤ 0.001a | ≤ 0.001a | ≤ 0.001a | 0.45a | ≤ 0.001a | ≤ 0.001a | ≤ 0.001a | ≤ 0.001b | 0.30b | 0.001a | ≤ 0.001a | 0.19b | ≤ 0.001a |
Average continental shale[47] WHO limits[48] USEPA limits[45] Dutch soil guidelines[49,50] Canadian soil guidelines[51] India (max; mg/kg), recycling site[12] China (mg/kg) burn site[52] Ghana (mean; mg/kg)[53] | 45 100 16 36 91 1,156 948 222 | 95 300 110 140 360 - 469 34 | 20 100 400 85 260 577 514 207 | 850 2,000 30 - - 4,675 - 46.8 | 68 50 - 35 50 157 27 7.6 | 90 100 25 100 87 219 61 3.32 | 0.4 - - 0.3 24 - 0.4 - | 130 - - - 130 - 69 - | 19 50 - - - 49 6.5 2.9 | 13 20 0.3 12 - 11.7 - | 0.3 3 0.6 0.8 22 179 2.29 0.53 | 0.6 - - - 2.9 - - - | 3 - - - - - - - |
Among the soil sampling sites, the highest mean concentrations of Pb (1,864 mg/kg), Cd (9.91 mg/kg), Cu (12,547 mg/kg), and Zn (1,444 mg/kg) were recorded at the combustion site, followed by disposal, dismantling, and storage site. In contrast, the mean concentrations of Hg were very high at the dismantling site (35 mg/kg), followed by disposal (32 mg/kg), storage (16 mg/kg), and combustion site (10 mg/kg) [Supplementary Table 2]. The maximum concentrations of Pb and Cu in this study were higher than the maximum concentrations recorded in the recycling site in India[12]. Evidence suggests that open burning of e-waste adversely impacts the environment by releasing toxic chemicals into the air, contaminating the soil where the burning occurs[59]. Moreover, runoff from areas contaminated with toxic metals from e-waste dumping sites can enter surrounding water bodies, elevating metal concentrations to levels that can make the water unsafe for aquatic animals[60]. It is important to note that many soil sampling sites were located near the river, increasing the likelihood of water contamination. These sites may require remedial action; co-contaminated soils can be effectively treated using physico-chemical, biochemical, and biological soil remediation approaches[61,62]. Given the large amount of e-waste being handled, more toxicological studies are needed; this could include examining a broad range of exposures from e-waste across diverse settings, incorporating longitudinal sample collection to measure exposure over time, and assessing the cumulative impact of multiple chemicals and pollutants. This approach would provide a more comprehensive picture of complex exposure profiles, enabling informed actions to reduce the release of harmful chemicals from unregulated e-waste recycling activities.
Trace element concentrations in dust
Similar to soil, exposed dust showed elevated Pb, Hg, Cu, and Zn levels, with mean concentrations of 439, 59, 617, and 1,202 mg/kg, respectively [Table 4]. Dust pollution was significantly higher at exposed sites compared to non-exposed sites (P ≤ 0.001), with concentrations approximately 30, 16, 32, and 6 times higher for Pb, Hg, Cu, and Zn, respectively. Additionally, Cr and Ni concentrations were significantly higher in exposed dust compared to non-exposed dust samples. This difference in toxic element concentrations between exposed and non-exposed control sites demonstrates the extent of contamination; however, including additional nearby contaminated sites would provide a clearer perspective on the full extent and spread of contamination. The trend of trace elements based on mean concentrations in exposed dust was Zn > Cu > Pb > Mn > Cr > Ni > Hg > V > Co > As > Cd > Se > Be. The concentrations of trace elements found in dust from this study align with findings from other e-waste sites in Bangladesh, China, and Egypt [Table 4]. For example, a similar study conducted in Dhaka, Bangladesh, which analyzed dust from three recycling shops, identified Pb and Cu as the most abundant elements, which is consistent with the current findings[57]. This highlights the distinct pollution patterns and contaminant profile associated with e-waste recycling. Notably, the dust Pb concentrations in this study were higher than those reported at other e-waste recycling sites[14,63,64], highlighting the high exposure risk for individuals. Multiple studies have demonstrated that dust exposure can significantly impact children living in e-waste recycling regions, as trace elements from e-waste are found in small particle sizes, which more easily affect child health[14,65]. Children exposed to dust at e-waste sites are at increased risk for health issues, including airway inflammation[14]. Although Cd levels were relatively modest in our study, earlier analyses have shown that increased dust Cd concentrations were associated with increased blood Cd levels among e-waste recycling workers[18]. We presume that uncontrolled e-waste recycling contributes to the widespread pollution of trace elements in surface dust, exposing workers to considerable health risks. Our findings highlight the role of dust as a vector for spreading trace elements in e-waste sites, creating potential exposure risks even beyond recycling facilities. Without appropriate controls, dust laden with trace elements may disperse into nearby communities, compounding environmental exposure for residents.
Trace element concentrations in dust (mg/kg)
Zn | Cu | Pb | Mn | Cr | Ni | Hg | V | Co | As | Cd | Se | Be | |
Exposed sites (n = 21) | |||||||||||||
Min | 199 | 81.88 | 58.63 | 145 | 14.47 | 13.50 | 3.64 | 8.33 | 0.00 | 1.95 | 0.47 | 0.11 | 0.18 |
Max | 4,495 | 1,886 | 923 | 1,436 | 849 | 185 | 562 | 21.69 | 32.28 | 7.06 | 9.18 | 0.54 | 0.51 |
50th percentile | 1,003 | 363 | 365 | 305 | 36.28 | 43.99 | 29.12 | 12.34 | 7.78 | 3.62 | 1.24 | 0.32 | 0.25 |
Average | 1,201 | 617 | 439 | 367 | 80.35 | 63.29 | 58.59 | 12.75 | 10.31 | 4.03 | 1.86 | 0.31 | 0.28 |
SD | 922 | 506 | 288 | 263 | 179 | 46.45 | 118 | 2.93 | 7.29 | 1.61 | 1.82 | 0.12 | 0.10 |
CV | 0.77 | 0.82 | 0.66 | 0.72 | 2.23 | 0.73 | 2.02 | 0.23 | 0.71 | 0.40 | 0.98 | 0.39 | 0.36 |
Non exposed sites (n = 10) | |||||||||||||
Min | 100 | 9.42 | 8.51 | 131 | 7.81 | 7.46 | 0.28 | 9.37 | 3.10 | 1.49 | 0.10 | 0.23 | 0.20 |
Max | 338 | 53.09 | 22.14 | 427 | 18.32 | 16.39 | 12.11 | 27.29 | 13.68 | 3.77 | 0.57 | 1.18 | 1.08 |
50th percentile | 220 | 13.00 | 14.97 | 226 | 13.18 | 11.18 | 2.14 | 14.54 | 4.68 | 2.23 | 0.13 | 0.42 | 0.33 |
Average | 213 | 19.11 | 14.60 | 243 | 12.47 | 11.76 | 3.56 | 15.97 | 5.60 | 2.28 | 0.18 | 0.47 | 0.40 |
SD | 82.11 | 13.96 | 3.54 | 86.61 | 3.33 | 3.67 | 3.36 | 5.23 | 3.24 | 0.73 | 0.14 | 0.27 | 0.25 |
CV | 0.39 | 0.73 | 0.24 | 0.36 | 0.27 | 0.31 | 0.95 | 0.33 | 0.58 | 0.32 | 0.81 | 0.59 | 0.62 |
P-value | ≤ 0.001a | ≤ 0.001a | ≤ 0.001a | 0.02a | ≤ 0.001a | ≤ 0.001a | ≤ 0.001a | 0.03b | 0.01a | 0.002b | ≤ 0.001a | 0.06a | 0.05a |
Bangladesh (max; mg/kg)[57] China (median; mg/kg)[14] China (mean; mg/kg)[63] Egypt (mean; mg/kg)[64] | - 1,072 - - | 9,585 402 - - | 10,105 145 399 311 | 1,998 493 - - | 122 147 151 - | 170 90.6 - - | - - - - | - 17.9 - - | - 7.34 - - | - 10.2 48.13 - | - 1.61 5.85 38.50 | - 4.48 - - | - - - - |
Igeo of soil and dust trace elements
We calculated the Igeo, which is useful for evaluating anthropogenic contamination of soils, sediment, and dust. Evidence suggests that the discharge of trace elements can impact the natural biological systems and disrupt the nutrient cycle in the environment[66,67]. The Igeo indicates that both soil and dust sampling sites were “uncontaminated” with Mn, Se, Be, and V in the e-waste exposed area [Figure 2]. However, soil from the e-waste sites was classified as “heavily to extremely contaminated” with Cd and Hg, with mean Igeo values of 4.01 and 4.88, respectively. Pb and Cu in exposed soil samples had mean Igeo values of 3.37 and 3.88, respectively, indicating “heavily contaminated”. Studies of e-waste processing sites in Nigeria have reported significant pollution with potentially toxic metals such as Cd, Pb, As, and Cu, with findings that are somewhat comparable to ours[68]. Dust samples were “heavily contaminated” with Pb and Cu, with mean Igeo values of 3.91 and 3.93, while “moderately to heavily contaminated” with Cd and Hg, with mean Igeo values of 2.45 and 2.37, respectively. Furthermore, the Igeo classified mean concentrations of Zn and Ni in soil and Cr and Ni in dust as “moderately contaminated”. Mowla et al. investigated three e-waste recycling shops in Dhaka, Bangladesh, and reported contamination levels based on individual shops. They found that Pb, Cu, and Ni posed a significantly higher contamination load compared to other measured metals, such as Mn and Cr[57]. The observed pollution index may not be entirely representative due to the lack of established regional background values in Bangladesh. However, we used reference values from non-exposed control sites, which could provide a somewhat reasonable estimate. The index suggests significant contamination of soils and dust with Pb, Cd, Hg, and Cu in the e-waste-exposed areas. We presume that the intensive, unregulated processing of e-waste has likely led to substantial metal releases into the environment, resulting in elevated trace element concentrations in soil and dust. Hence, it ought to be concerned about the trace element pollution at the e-waste site.
Non-carcinogenic risks
Table 5 illustrates the HI results from three pathways for soil and dust. Since trace element concentrations are not normally distributed, the median, maximum, and minimum HQ for ingestion, inhalation, and dermal exposures, as well as the absolute HI values, are presented in Supplementary Tables 3 and 4 (soil) and Supplementary Tables 5 and 6 (dust). The results indicate that ingestion appears to be the primary pathway for Pb, Hg, and Cu exposure from soil and Pb, Hg, and Cr exposure from dust. Previous studies have also highlighted non-dietary ingestion as the most significant exposure pathway in terms of health risks. In contrast, dermal contact and inhalation routes through soil and dust were almost negligible, consistent with findings from studies conducted elsewhere[69,70]. For most trace elements, the HQ values for soil exposure were slightly higher than those for dust exposure, primarily due to higher rates of soil ingestion compared to dust ingestion.
Median values of HQ of toxic metals through ingestion, inhalation and dermal contact and HI values of soil and dust to an exposed child and adult in Bangladesh
HQingestion | HQinhalation | HQdermal | HI | |||||
Child | Adult | Child | Adult | Child | Adult | Child | Adult | |
E-waste-exposed soil | ||||||||
Pb | 1.15 × 10+00 | 1.24 × 10-01 | 3.20 × 10-05 | 1.81 × 10-05 | 2.15 × 10-02 | 3.29 × 10-02 | 1.18 | 0.16 |
Cd | 2.01 × 10-02 | 2.15 × 10-03 | 5.61 × 10-07 | 3.16 × 10-07 | 5.62 × 10-03 | 8.59 × 10-03 | 0.03 | 0.01 |
Hg | 4.56 × 10-01 | 4.89 × 10-02 | 1.27 × 10-05 | 7.18 × 10-06 | 1.60 × 10-02 | 2.44 × 10-02 | 0.47 | 0.07 |
As | 1.42 × 10-01 | 1.52 × 10-02 | 9.67 × 10-06 | 5.45 × 10-06 | 3.97 × 10-04 | 6.07 × 10-04 | 0.14 | 0.02 |
Cr | 1.19 × 10-01 | 1.28 × 10-02 | 3.49 × 10-04 | 1.97 × 10-04 | 1.67 × 10-02 | 2.55 × 10-02 | 0.14 | 0.04 |
Cu | 1.49 × 10-01 | 1.60 × 10-02 | 3.84 × 10-06 | 2.16 × 10-06 | 1.29 × 10-03 | 1.97 × 10-03 | 0.15 | 0.02 |
Mn | 2.05 × 10-02 | 2.19E-03 | 5.60 × 10-03 | 3.16 × 10-03 | 4.36 × 10-03 | 6.65 × 10-03 | 0.03 | 0.01 |
Ni | 1.71 × 10-02 | 1.83 × 10-03 | 6.69 × 10-04 | 3.77 × 10-04 | 1.78 × 10-04 | 2.71 × 10-04 | 0.02 | 0.00 |
Zn | 2.90 × 10-02 | 3.11 × 10-03 | 8.11 × 10-07 | 4.57 × 10-07 | 4.06 × 10-04 | 6.20 × 10-04 | 0.03 | 0.00 |
E-waste-exposed dust | ||||||||
Pb | 1.33 × 10+00 | 1.43 × 10-01 | 3.70 × 10-05 | 2.09 × 10-05 | 2.49 × 10-02 | 3.79 × 10-02 | 1.36 | 0.18 |
Cd | 1.59 × 10-02 | 1.70 × 10-03 | 4.44 × 10-07 | 2.50 × 10-07 | 4.45 × 10-03 | 6.79 × 10-03 | 0.02 | 0.01 |
Hg | 1.24 × 10+00 | 1.33 × 10-01 | 3.47 × 10-05 | 1.96 × 10-05 | 4.34 × 10-02 | 6.63 × 10-02 | 1.28 | 0.20 |
As | 1.54 × 10-01 | 1.65 × 10-02 | 1.05 × 10-05 | 5.92 × 10-06 | 4.31 × 10-04 | 6.59 × 10-04 | 0.15 | 0.02 |
Cr | 1.55 × 10-01 | 1.66 × 10-02 | 4.53 × 10-04 | 2.56 × 10-04 | 2.16 × 10-02 | 3.30 × 10-02 | 0.18 | 0.05 |
Cu | 1.26E-01 | 1.35 × 10-02 | 3.23E-06 | 1.82 × 10-06 | 1.08 × 10-03 | 1.66 × 10-03 | 0.13 | 0.02 |
Mn | 2.79 × 10-02 | 2.98 × 10-03 | 7.62 × 10-03 | 4.30 × 10-03 | 5.93 × 10-03 | 9.06 × 10-03 | 0.04 | 0.02 |
Ni | 2.81 × 10-02 | 3.01 × 10-03 | 1.10 × 10-03 | 6.20 × 10-04 | 2.92 × 10-04 | 4.45 × 10-04 | 0.03 | 0.00 |
Zn | 4.27 × 10-02 | 4.58 × 10-03 | 1.19 × 10-06 | 6.73 × 10-07 | 5.98 × 10-04 | 9.14 × 10-04 | 0.04 | 0.01 |
Exposed soil Pb presented non-carcinogenic ingestion risks to children, with a median HQ of 1.15 and an overall HI value of 1.18 from all three pathways [Table 5 and Supplementary Table 3]. The HQ values for maximum Pb and Cu concentrations in exposed soil indicated a non-carcinogenic ingestion risk for both children and adults [Supplementary Table 3]. Notably, the calculated HI values for children were significantly higher (Pb: 15.60, Cu: 10.23) than for adults (Pb: 2.08, Cu: 1.22), consistent with other studies showing higher non-carcinogenic risks during childhood than adulthood[71,72]. Maximum soil Hg posed a non-carcinogenic ingestion risk (HQ 5.25) for children only (HI: 5.44); the median concentration did not pose a risk to either children or adults (< 1). For both children and adults, the HQ and HI values for the dermal and inhalation pathways were below 1, indicating no significant risk from these routes of exposure. In contrast, Cd, As, Ni, Zn, and Mn did not present any non-carcinogenic risks for either children or adults from exposed soil exposure [Supplementary Table 3]. No elements in the non-exposed control sites posed non-carcinogenic risks to children or adults, as the minimum, maximum, and median HQ values for all three pathways remained below 1 for these trace elements [Supplementary Table 4].
The potential risks of trace element exposure from dust ingestion were relatively higher for children, with a median HQ of 1.33 for Pb and 1.24 for Hg. The calculated HI values for Pb and Hg were 1.36 and 1.28, respectively [Table 5 and Supplementary Table 5]. These findings are consistent with studies indicating significant non-carcinogenic risks from dust ingestion in e-waste recycling regions, highlighting a substantial risk for children exposed to dust trace elements through ingestion[9,14]. Maintaining adequate ventilation and routinely cleaning surfaces could possibly reduce the amount of dust consumption by children. In contrast, dust inhalation and dermal contact posed no significant risk for children or adults. The median HI values for Cd, As, Cr, Cu, Mn, Ni, and Zn in dust were 0.02, 0.15, 0.18, 0.13, 0.04, 0.01, and 0.04 for children and 0.01, 0.02, 0.05, 0.01, 0.02. 0.00, and 0.01 for adults, considering all three pathways
Carcinogenic risks
We calculated the cancer risk for children and adults from each element in the soil and dust considering ingestion, inhalation, and dermal pathways; a detailed analysis is presented in Supplementary Tables 7-10. Some trace elements were excluded from this analysis due to the lack of CSF values. For both exposed children and adults, the median values of carcinogenic agents from soil and dust samples were lower than the permissible limit of 1 × 10-6 to 1 × 10-4. For adults, the calculated TCR from the three pathways for Pb, Cd, As, Cr, and Ni in exposed soils were 1.51 × 10-6, 2.81 × 10-7, 2.36 × 10-6, 6.75 × 10-6, and 1.17 × 10-5, respectively. For children, the corresponding values were 3.35 × 10-6, 6.54 × 10-7, 5.49 × 10-6, 1.55 × 10-5, and 2.71 × 10-5 for Pb, Cd, As, Cr, and Ni, respectively [Table 6 and Supplementary Table 7]. However, the TCR value for the maximum concentration of soil Ni was 1.21 × 10-4, indicating a potential cancer risk for exposed children [Supplementary Table 7]. Evidence suggests that humans are highly susceptible to carcinogen nickel, which can enter the body through the skin, digestive system, and respiratory passages, with nasal and lung cancers closely correlated with Ni exposure[73,74].
Median values of CR of toxic metals through ingestion, inhalation and dermal contact and TCR values of soil and dust to an exposed child and adult in Bangladesh
CRingestion | CRinhalation | CRdermal | TCR | |||||
Child | Adult | Child | Adult | Child | Adult | Child | Adult | |
E-waste-exposed soil | ||||||||
Pb | 2.94 × 10-06 | 1.26 × 10-06 | 4.06 × 10-10 | 9.16 × 10-10 | 4.12 × 10-07 | 2.52 × 10-07 | 3.35 × 10-06 | 1.51 × 10-06 |
Cd | 6.54 × 10-07 | 2.80 × 10-07 | 3.03 × 10-10 | 6.83 × 10-10 | 0.00 × 10+00 | 0.00 × 10+00 | 6.54 × 10-07 | 2.81 × 10-07 |
As | 5.47 × 10-06 | 2.35 × 10-06 | 1.54 × 10-09 | 3.47 × 10-09 | 1.53 × 10-08 | 9.36 × 10-09 | 5.49 × 10-06 | 2.36 × 10-06 |
Cr | 1.53 × 10-05 | 6.56 × 10-06 | 3.51 × 10-08 | 7.91 × 10-08 | 1.80 × 10-07 | 1.10 × 10-07 | 1.55 × 10-05 | 6.75 × 10-06 |
Ni | 2.67 × 10-05 | 1.14 × 10-05 | 6.89 × 10-10 | 1.55 × 10-09 | 3.74 × 10-07 | 2.28 × 10-07 | 2.71 × 10-05 | 1.17 × 10-05 |
E-waste-exposed dust | ||||||||
Pb | 3.40 × 10-06 | 1.46 × 10-06 | 4.69 × 10-10 | 1.06 × 10-09 | 4.75 × 10-07 | 2.90 × 10-07 | 3.87 × 10-06 | 1.75 × 10-06 |
Cd | 5.18 × 10-07 | 2.22 × 10-07 | 2.40 × 10-10 | 5.41 × 10-10 | 0.00 × 10+00 | 0.00 × 10+00 | 5.18 × 10-07 | 2.22 × 10-07 |
As | 5.94 × 10-06 | 2.55 × 10-06 | 1.67 × 10-09 | 3.77 × 10-09 | 1.66 × 10-08 | 1.02 × 10-08 | 5.96 × 10-06 | 2.56 × 10-06 |
Cr | 1.99 × 10-05 | 8.52 × 10-06 | 4.55 × 10-08 | 1.03 × 10-07 | 2.34 × 10-07 | 1.43 × 10-07 | 2.02 × 10-06 | 8.76 × 10-06 |
Ni | 4.39 × 10-05 | 1.88 × 10-05 | 1.13 × 10-09 | 2.55 × 10-09 | 6.14 × 10-07 | 3.75 × 10-07 | 4.45 × 10-06 | 1.92 × 10-06 |
For dust exposure, the carcinogenic risk calculated for median concentrations of Pb, Cd, As, Cr, and Ni for adults were 1.75 × 10-6, 2.22 × 10-7, 2.56 × 10-6, 8.76 × 10-6 and 1.92 × 10-5, respectively. Comparable risks for children were 3.87 × 10-6, 5.18 × 10-7, 5.96 × 10-6, 2.02 × 10-5 and 4.45 × 10-5, respectively [Table 6 and Supplementary Table 8]. In contrast, the TCR values for the maximum concentration of Cr in dust were significantly higher than the target value, with TCR of 4.72 × 10-4 for children and 2.05 × 10-4 for adults. Cr has been identified as a major contributor to the carcinogenic effects of dust pollution in several studies[75,76]. Given that Cr is prevalent in the environment, exposure to this metal can lead to carcinogenic consequences including lung cancer, nose and nasal sinus cancer, and suspected laryngeal and stomach cancers[77]. Similarly, the TCR for the maximum Ni concentration in dust for children exceeded the target value of 1 × 10-4, indicating that children may develop cancer from ingesting Ni-contaminated dust, while the TCR for adults was below the target value [Supplementary Table 8]. Similar to non-carcinogenic risks, ingestion is the main route of exposure for carcinogenicity, particularly in children due to their lower BW and higher rates of dust ingestion[78]. The CR in our study was primarily attributed to exposure to Cr and Ni, making these high-priority metals that require control measures to mitigate their carcinogenic effects. It is important to note that the TCR is likely elevated at contaminated sites with high concentrations of Ni in soil and elevated levels of Cr and Ni in dust. In contrast, non-exposed soil and dust did not show any carcinogenic effects for either children or adults [Supplementary Tables 9 and 10]. Given the increasing e-waste recycling activities and rapid urbanization, there is a possibility that carcinogenic risks from other elements will exceed safe levels in the future. This highlights the need for increased attention to the potential carcinogenic risks to children caused by e-waste recycling and other industrial activities. We assume that the cancer risk assessed using trace element concentrations from soil and dust may have either exaggerated or underestimated the actual health risk compared to using bioavailable trace metal concentrations[79]. Nevertheless, the findings from this study provide valuable data that can aid authorities in assessing trace element pollution levels in the exposed area.
Uncertainty of risk analysis, limitations and strengths
Human health risk assessment is inherently uncertain; thus, the possibility of uncertainties in this work exists and could be considered a limitation on the applicability of the risk estimation. First, the probability factors derived from the US-EPA guidelines for estimation may exhibit significant regional or individual differences, leading to uncertainty in the risk analysis. However, to reduce the uncertainty, we used a few country-specific parameters that could mitigate some variation. Second, the EPA’s health risk model evaluates the toxicity of individual trace elements without considering the potential cumulative effects of multiple exposures. Soil and dust samples may also contain other chemicals, such as POPs, flame retardants, and plasticizers from recycling activities, which were not evaluated in the studied samples. This can result in an underestimation of risk, particularly in situations where individuals are exposed to multiple chemicals or pollutants at the same time. Thus, we assume that the actual risk of exposure to soil and dust may be greater than the predicted estimates in this study. Future studies should investigate the health risks posed by other substances released during e-waste recycling activities.
Third, the ingestion of trace metals through the dietary route was not considered when interpreting the findings. If dietary intake had been included, the CDI of trace elements would have been higher, likely resulting in greater estimated risk. Failing to account for dietary intake likely leads to bias and risk underestimation. Fourth, cancer risk estimates in this study are also thought to be lower than the true risk. The US-EPA usually determines SFs to potential carcinogens categorized as class A, B1, and B2, which refer to human carcinogen, probable human carcinogen with limited human data, and probable human carcinogen with adequate evidence from animal studies but insufficient or no human data[45]. This could potentially bias the estimation. Finally, EPA models might not fully account for the possible health impacts on vulnerable populations, such as children, pregnant women, or those with pre-existing diseases. These populations may require additional attention in health risk assessment. We collected the samples during the COVID-19 pandemic when the supply chain for e-waste was disrupted, the scope of the job was limited, and there was less recycled e-waste. These factors might have limited exposure to the environment, which could reduce the observed risk.
However, despite these constraints and uncertainties, the use of a standardized and transparent approach allowed us to draw reasonable conclusions by comparing the data from exposed sites to non-exposed sites, where contamination levels remained below the threshold. Although this risk assessment might not provide a completely accurate estimation of the amount of risk, it provides an overall evaluation of the health risk associated with toxic metal exposure resulting from soil and dust in e-waste exposed and non-exposed areas of Bangladesh. This scientifically rigorous and reliable model is designed to facilitate risk communication and help stakeholders understand the potential health risks associated with environmental exposures.
CONCLUSIONS
We evaluated the concentrations of 13 trace elements, pollution levels, and consequent health risk assessment in soil and dust from e-waste exposed and non-exposed sites. Our findings unequivocally showed increased concentrations of Pb, Hg, Cu, and Zn in the vicinity of e-waste-exposed soil and dust compared to non-exposed soil and dust, which could lead to a myriad of health and environmental problems. The concentrations of these metals in contaminated soil and dust exceeded the permissible limit set by WHO and USEPA standards. The health risk assessment model indicated that ingestion is the dominant exposure pathway, particularly for children, who are more vulnerable to exposed trace elements from soil and dust. Evidence suggests that children are more vulnerable to a particular dosage of the harmful substances due to their immature developing metabolic systems, pica behavior, and the tendency for finger-licking[80]. We identified a notable non-carcinogenic risk, primarily from exposure to Pb and Cu in soil and Pb and Hg in dust. The carcinogenic risks of Cr and Ni were found to be intolerable at very high concentrations.
This study highlights significant areas of vulnerability and pollution associated with trace metal exposure. To combat the negative consequences of primitive e-waste recycling activities in Bangladesh, the “Hazardous Waste (e-waste) Management Rules” were recently introduced[81]. These regulations aim to protect both the environment and human health; however, effective implementation will require a strategic approach to ensure environmental protection and compliance. Substantial efforts are required to restrict the release of toxic elements and deploy cost-effective interventions to reduce soil and dust-related trace metal exposure in e-waste environments. Moreover, there is an urgent need to set environmental contamination standards in Bangladesh. Employers in the e-waste recycling sector should be positive in educating workers about these risks and ensuring the availability of appropriate protective equipment during recycling. Additionally, implementing effective control measures is essential to protect workers from direct exposure and mitigate indirect exposure to nearby populations, thereby reducing the health risks associated with environmental pollution.
DECLARATIONS
Acknowledgments
The authors thank the participants who allowed sample collection at the study sites. The authors also thank the research team for their invaluable cooperation throughout the sample collection process. icddr,b is also grateful to the Governments of Bangladesh and Canada for providing core/unrestricted support.
Authors’ contributions
Conceptualization: Parvez SM
Funding acquisition: Parvez SM
Data curation: Parvez SM, Jahan F, Hares A, Hasan SS, Aich N, Islam N
Formal analysis: Parvez SM, Hares A, Hasan SS
Investigation: Parvez SM, Jahan F, Hares A, Hasan SS, Rahman M, Aich N, Islam N, Islam Z
Methodology: Parvez SM, Sly PD, Raqib R, Hasan SS
Project administration: Parvez SM, Jahan F, Hares A, Hasan SS, Rahman M
Laboratory analysis: Moniruzzaman M, Parvin A
Visualization: Parvez SM, Hares A, Hasan SS
Writing - original draft preparation: Parvez SM
Writing - review and editing: Parvez SM, Hasan SS, Moniruzzaman M, Jahan F, Rahman M, Aich N, Islam N, Hares A, Parvin A, Islam Z, Raqib R, Knibbs LD, Sly PD
Availability of data and materials
All data are included in the manuscript and can be found in the Supplementary Materials.
Financial support and sponsorship
This research study was funded by the Swedish International Development Cooperation Agency (Sida) (Grant no. 54100089). icddr,b and the University of Queensland acknowledge Sida for its research efforts.
Conflicts of interest
All authors declared that there are no conflicts of interest.
Ethical approval and consent to participate
This study protocol has been reviewed and approved by the Research Review Committee and Ethical Review Committee of the icddr,b (PR#19057) and The University of Queensland’s Human Behavioral Ethics Committee (2021/HE001648).
Consent for publication
Not applicable.
Copyright
© The Author(s) 2024.
Supplementary Materials
REFERENCES
1. Heacock M, Kelly CB, Suk WA. E-waste: the growing global problem and next steps. Rev Environ Health 2016;31:131-5.
2. Forti V, Baldé CP, Kuehr R, Bel G. The global e-waste monitor 2020. Available from: https://ewastemonitor.info/wp-content/uploads/2020/11/GEM_2020_def_july1_low.pdf. [Last accessed on 11 Dec 2024].
3. Parvez SM, Jahan F, Brune MN, et al. Health consequences of exposure to e-waste: an updated systematic review. Lancet Planet Health 2021;5:e905-20.
4. Grant K, Goldizen FC, Sly PD, et al. Health consequences of exposure to e-waste: a systematic review. Lancet Glob Health 2013;1:e350-61.
5. Zhuang X. Chapter 14 - Chemical hazards associated with treatment of waste electrical and electronic equipment. In: Electronic waste management and treatment technology. Elsevier; 2019. pp. 311-34.
6. Chen Y, Li J, Liu L, Zhao N. Polybrominated diphenyl ethers fate in China: a review with an emphasis on environmental contamination levels, human exposure and regulation. J Environ Manage 2012;113:22-30.
7. Li J, Lu H, Guo J, Xu Z, Zhou Y. Recycle technology for recovering resources and products from waste printed circuit boards. Environ Sci Technol 2007;41:1995-2000.
8. Leung A, Cai ZW, Wong MH. Environmental contamination from electronic waste recycling at Guiyu, southeast China. J Mater Cycles Waste Manag 2006;8:21-33.
9. Zheng J, Chen KH, Yan X, et al. Heavy metals in food, house dust, and water from an e-waste recycling area in South China and the potential risk to human health. Ecotoxicol Environ Saf 2013;96:205-12.
10. Pradhan JK, Kumar S. Informal e-waste recycling: environmental risk assessment of heavy metal contamination in Mandoli industrial area, Delhi, India. Environ Sci Pollut Res Int 2014;21:7913-28.
11. Song Q, Li J. Environmental effects of heavy metals derived from the e-waste recycling activities in China: a systematic review. Waste Manag 2014;34:2587-94.
12. Dutta D, Goel S, Kumar S. Health risk assessment for exposure to heavy metals in soils in and around E-waste dumping site. J Environ Chem Eng 2022;10:107269.
13. Tran TS, Dinh VC, Nguyen TAH, Kim KW. Soil contamination and health risk assessment from heavy metals exposure near mining area in Bac Kan province, Vietnam. Environ Geochem Health 2022;44:1189-202.
14. Xu R, Zheng X, Lin Y, Lin C, Guo Y, Huo X. Assessment of dust trace elements in an e-waste recycling area and related children’s health risks. Sci Total Environ 2021;791:148154.
15. Centre for Environmental and Resource Management. Assessment of generation of e-waste, its impacts on environment and resource recovery potential in Bangladesh. 2018. Available from: https://doe.portal.gov.bd/sites/default/files/files/doe.portal.gov.bd/page/1f58f60a_51d9_46c0_9fa1_79b7b565db05/2020-10-01-13-02-e522e1499ac288d119a6f7ae16c7f7d0.pdf. [Last accessed on 11 Dec 2024].
16. Mahmud I, Sultana S, Rahman A, Ramayah T, Cheng Ling T. E-waste recycling intention paradigm of small and medium electronics store managers in Bangladesh: an S-O-R perspective. Waste Manag Res 2020;38:1438-49.
17. Chan JK, Wong MH. A review of environmental fate, body burdens, and human health risk assessment of PCDD/Fs at two typical electronic waste recycling sites in China. Sci Total Environ 2013;463-4:1111-23.
18. Parvez SM, Jahan F, Abedin J, et al. Blood lead, cadmium and hair mercury concentrations and association with soil, dust and occupational factors in e-waste recycling workers in Bangladesh. Int J Hyg Environ Health 2024;257:114340.
19. Parvez SM, Hasan SS, Knibbs LD, et al. Ecological burden of e-waste in Bangladesh - an assessment to measure the exposure to e-waste and associated health outcomes: protocol for a cross-sectional study. JMIR Res Protoc 2022;11:e38201.
20. Moniruzzaman M, Shaikh MAA, Saha B, Shahrukh S, Jawaa ZT, Khan MF. Seasonal changes and respiratory deposition flux of PM2.5 and PM10 bound metals in Dhaka, Bangladesh. Chemosphere 2022;309:136794.
21. Müller G. Index of geoaccumulation in sediments of the Rhine River. J Geol 1969;2:108-18. Available from: https://www.scienceopen.com/document?vid=4b875795-5729-4c05-9813-64951e2ca488. [Last accessed on 11 Dec 2024]
22. Li Z, Feng X, Li G, et al. Distributions, sources and pollution status of 17 trace metal/metalloids in the street dust of a heavily industrialized city of central China. Environ Pollut 2013;182:408-16.
23. USEPA. Update for Chapter 5 of thE Exposure Factors Handbook. Soil and dust ingestion. EPA/600/R-17/384F. 2017. Available from: https://www.epa.gov/sites/default/files/2018-01/documents/efh-chapter05_2017.pdf. [Last accessed on 11 Dec 2024].
24. Liu X, Song Q, Tang Y, et al. Human health risk assessment of heavy metals in soil-vegetable system: a multi-medium analysis. Sci Total Environ 2013;463-4:530-40.
25. Wang X, Sato T, Xing B, Tao S. Health risks of heavy metals to the general public in Tianjin, China via consumption of vegetables and fish. Sci Total Environ 2005;350:28-37.
26. USEPA. Exposure Factors Handbook (1997, final report). EPA/600/P-95/002F a-c. 1997. Available from: https://cfpub.epa.gov/ncea/risk/recordisplay.cfm?deid=12464. [Last accessed on 11 Dec 2024].
27. Trojanowska M, Świetlik R. Investigations of the chemical distribution of heavy metals in street dust and its impact on risk assessment for human health, case study of Radom (Poland). Hum Ecol Risk Assess 2020;26:1907-26.
28. Gupta V, Bisht L, Arya AK, Singh AP, Gautam S. Spatially resolved distribution, sources, exposure levels, and health risks of heavy metals in <63 μm size-fractionated road dust from Lucknow City, North India. Int J Environ Res Public Health 2022;19:12898.
29. Zhang J, Wu L, Zhang Y, Li F, Fang X, Mao H. Elemental composition and risk assessment of heavy metals in the PM10 fractions of road dust and roadside soil. Particuology 2019;44:146-52.
30. USEPA. Risk Assessment Guidance for Superfund Volume I: human health evaluation manual (Part E, Supplemental Guidance for Dermal Risk Assessment). 2004. Available from: https://www.epa.gov/sites/default/files/2015-09/documents/part_e_final_revision_10-03-07.pdf. [Last accessed on 11 Dec 2024].
31. Rakib MRJ, Jolly YN, Enyoh CE, et al. Levels and health risk assessment of heavy metals in dried fish consumed in Bangladesh. Sci Rep 2021;11:14642.
32. Akter A, Hosen A, Hossain MA, Khalil F, Mustafa T. Heavy metal concentrations and human health risk assessment of selected wild and cultured fishes of Bangladesh. Bangladesh J Zool 2021;49:189-203.
33. Duan Z, Wang J, Cai X, Wu Y, Xuan B. Spatial distribution and human health risk assessment of heavy metals in campus dust: a case study of the university town of Huaxi. Hum Ecol Risk Assess 2020;26:986-99.
34. Musa AA, Hamza SM, Kidak R. Street dust heavy metal pollution implication on human health in Nicosia, North Cyprus. Environ Sci Pollut Res Int 2019;26:28993-9002.
35. Ghanavati N, Nazarpour A, De Vivo B. Ecological and human health risk assessment of toxic metals in street dusts and surface soils in Ahvaz, Iran. Environ Geochem Health 2019;41:875-91.
36. Hini G, Eziz M, Wang W, Ili A, Li X. Spatial distribution, contamination levels, sources, and potential health risk assessment of trace elements in street dusts of Urumqi city, NW China. Hum Ecol Risk Assess 2020;26:2112-28.
37. Wei X, Gao B, Wang P, Zhou H, Lu J. Pollution characteristics and health risk assessment of heavy metals in street dusts from different functional areas in Beijing, China. Ecotoxicol Environ Saf 2015;112:186-92.
38. Qadeer A, Saqib ZA, Ajmal Z, et al. Concentrations, pollution indices and health risk assessment of heavy metals in road dust from two urbanized cities of Pakistan: Comparing two sampling methods for heavy metals concentration. Sustain Cities Soc 2020;53:101959.
39. USEPA. Supplemental Guidance for developing soil screening levels for superfund sites. 2002. Available from: https://semspub.epa.gov/work/HQ/175878.pdf. [Last accessed on 11 Dec 2024].
40. Roy S, Gupta SK, Prakash J, Habib G, Baudh K, Nasr M. Ecological and human health risk assessment of heavy metal contamination in road dust in the National Capital Territory (NCT) of Delhi, India. Environ Sci Pollut Res Int 2019;26:30413-25.
41. Idris AM, Alqahtani FM, Said TO, Fawy KF. Contamination level and risk assessment of heavy metal deposited in street dusts in Khamees-Mushait city, Saudi Arabia. Hum Ecol Risk Assess 2020;26:495-511.
42. Li H, Qian X, Hu W, Wang Y, Gao H. Chemical speciation and human health risk of trace metals in urban street dusts from a metropolitan city, Nanjing, SE China. Sci Total Environ 2013;456-7:212-21.
43. Li F, Zhang J, Huang J, et al. Heavy metals in road dust from Xiandao District, Changsha City, China: characteristics, health risk assessment, and integrated source identification. Environ Sci Pollut Res Int 2016;23:13100-13.
44. USEPA. Integrated Risk Information System (IRIS). Available from: https://www.epa.gov/iris. [Last accessed on 11 Dec 2024].
45. USEPA. Guidelines for carcinogen risk assessment. EPA/630/P-03/001F. 2005. Available from: https://www.epa.gov/sites/default/files/2013-09/documents/cancer_guidelines_final_3-25-05.pdf. [Last accessed on 11 Dec 2024].
46. Stewart BW, Wild CP. World Cancer Report 2014. Available from: https://publications.iarc.fr/Non-Series-Publications/World-Cancer-Reports/World-Cancer-Report-2014. [Last accessed on 11 Dec 2024]
47. Turekian KK, Wedepohl KH. Distribution of the elements in some major units of the earth’s crust. Geol Soc America Bull 1961;72:175.
48. Kinuthia GK, Ngure V, Beti D, Lugalia R, Wangila A, Kamau L. Levels of heavy metals in wastewater and soil samples from open drainage channels in Nairobi, Kenya: community health implication. Sci Rep 2020;10:8434.
49. Vodyanitskii Y. Standards for the contents of heavy metals in soils of some states. Ann Agrar Sci 2016;14:257-63.
50. Crommentuijn T, Sijm D, de Bruijn J, van den Hoop M, van Leeuwen K, van de Plassche E. Maximum permissible and negligible concentrations for metals and metalloids in the Netherlands, taking into account background concentrations. J Environ Manage 2000;60:121-43.
51. Canadian Council of Ministers of the Environment. Canadian soil quality guidelines for the protection of environmental and human health: summary tables. 2007. Available from: https://support.esdat.net/Environmental%20Standards/canada/soil/rev_soil_summary_tbl_7.0_e.pdf. [Last accessed on 11 Dec 2024].
52. Liu M, Huang B, Bi X, Ren Z, Sheng G, Fu J. Heavy metals and organic compounds contamination in soil from an e-waste region in South China. Environ Sci Processes Impacts 2013;15:919-29.
53. Akortia E, Olukunle OI, Daso AP, Okonkwo JO. Soil concentrations of polybrominated diphenyl ethers and trace metals from an electronic waste dump site in the Greater Accra Region, Ghana: implications for human exposure. Ecotoxicol Environ Saf 2017;137:247-55.
54. Arya S, Rautela R, Chavan D, Kumar S. Evaluation of soil contamination due to crude E-waste recycling activities in the capital city of India. Process Saf Environ 2021;152:641-53.
55. Parvez SM, Huda MM, Rahman M, et al. Hormonal, liver, and renal function associated with electronic waste (e-waste) exposure in Dhaka, Bangladesh. Toxicology 2024;505:153833.
56. Parvez SM, Huda MM, Rahman M, et al. Hematological, cardiovascular and oxidative DNA damage markers associated with heavy metal exposure in electronic waste (e-waste) workers of Bangladesh. Toxicology 2024;509:153978.
57. Mowla M, Rahman E, Islam N, Aich N. Assessment of heavy metal contamination and health risk from indoor dust and air of informal E-waste recycling shops in Dhaka, Bangladesh. J Hazard Mater 2021;4:100025.
58. Zhang XL, Luo XJ, Liu HY, Yu LH, Chen SJ, Mai BX. Bioaccumulation of several brominated flame retardants and dechlorane plus in waterbirds from an e-waste recycling region in South China: associated with trophic level and diet sources. Environ Sci Technol 2011;45:400-5.
59. Alcántara-Concepción V, Gavilán-García A, Gavilán-García IC. Environmental impacts at the end of life of computers and their management alternatives in México. J Clean Prod 2016;131:615-28.
60. Olafisoye OB, Adefioye T, Osibote OA. Heavy metals contamination of water, soil, and plants around an electronic waste dumpsite. Pol J Environ Stud 2013;22:1431-9. Available from: https://www.researchgate.net/profile/Otolorin-Osibote-2/publication/281446780_Heavy_Metals_Contamination_of_Water_Soil_and_Plants_around_an_Electronic_Waste_Dumpsite/links/55e7fe0c08ae21d099c15e9c/Heavy-Metals-Contamination-of-Water-Soil-and-Plants-around-an-Electronic-Waste-Dumpsite.pdf. [Last accessed on 11 Dec 2024]
61. Ye S, Zeng G, Wu H, et al. Biological technologies for the remediation of co-contaminated soil. Crit Rev Biotechnol 2017;37:1062-76.
62. Ye S, Zeng G, Wu H, et al. Co-occurrence and interactions of pollutants, and their impacts on soil remediation - a review. Crit Rev Env Sci Tec 2017;47:1528-53.
63. Wu Y, Li Y, Kang D, et al. Tetrabromobisphenol A and heavy metal exposure via dust ingestion in an e-waste recycling region in Southeast China. Sci Total Environ 2016;541:356-64.
64. Khoder M, Hassan S, El-abssawy A. An evaluation of loading rate of dust, Pb, Cd, and Ni and metals mass concentration in the settled surface dust in domestic houses and factors affecting them. Indoor Built Environ 2010;19:391-9.
65. Huang CL, Bao LJ, Luo P, Wang ZY, Li SM, Zeng EY. Potential health risk for residents around a typical e-waste recycling zone via inhalation of size-fractionated particle-bound heavy metals. J Hazard Mater 2016;317:449-56.
66. Nowrouzi M, Pourkhabbaz A. Application of geoaccumulation index and enrichment factor for assessing metal contamination in the sediments of Hara Biosphere Reserve, Iran. Chem Spec Bioavailab 2014;26:99-105.
67. Luo C, Liu C, Wang Y, et al. Heavy metal contamination in soils and vegetables near an e-waste processing site, South China. J Hazard Mater 2011;186:481-90.
68. Adenuga AA, Amos OD, Olajide OD, Eludoyin AO, Idowu OO. Environmental impact and health risk assessment of potentially toxic metals emanating from different anthropogenic activities related to E-wastes. Heliyon 2022;8:e10296.
69. Tao XQ, Shen DS, Shentu JL, Long YY, Feng YJ, Shen CC. Bioaccessibility and health risk of heavy metals in ash from the incineration of different e-waste residues. Environ Sci Pollut Res Int 2015;22:3558-69.
70. Ackah M. Soil elemental concentrations, geoaccumulation index, non-carcinogenic and carcinogenic risks in functional areas of an informal e-waste recycling area in Accra, Ghana. Chemosphere 2019;235:908-17.
71. Masri S, LeBrón AMW, Logue MD, et al. Risk assessment of soil heavy metal contamination at the census tract level in the city of Santa Ana, CA: implications for health and environmental justice. Environ Sci Process Impacts 2021;23:812-30.
72. Chonokhuu S, Batbold C, Chuluunpurev B, Battsengel E, Dorjsuren B, Byambaa B. Contamination and health risk assessment of heavy metals in the soil of major cities in Mongolia. Int J Environ Res Public Health 2019;16:2552.
73. Zdrojewicz Z, Popowicz E, Winiarski J. Nickel - role in human organism and toxic effects. Pol Merkur Lekarski 2016;41:115-8.
74. Son YO. Molecular mechanisms of nickel-induced carcinogenesis. Endocr Metab Immune Disord Drug Targets 2020;20:1015-23.
75. Madadi R, Mohamadi S, Rastegari M, et al. Health risk assessment and source apportionment of potentially toxic metal(loid)s in windowsill dust of a rapidly growing urban settlement, Iran. Sci Rep 2022;12:19736.
76. Long Z, Zhu H, Bing H, et al. Contamination, sources and health risk of heavy metals in soil and dust from different functional areas in an industrial city of Panzhihua City, Southwest China. J Hazard Mater 2021;420:126638.
77. den Braver-Sewradj SP, van Benthem J, Staal YCM, Ezendam J, Piersma AH, Hessel EVS. Occupational exposure to hexavalent chromium. Part II. Hazard assessment of carcinogenic effects. Regul Toxicol Pharmacol 2021;126:105045.
78. Gul HK, Gullu G, Babaei P, Nikravan A, Kurt-Karakus PB, Salihoglu G. Assessment of house dust trace elements and human exposure in Ankara, Turkey. Environ Sci Pollut Res Int 2023;30:7718-35.
79. Oomen AG, Hack A, Minekus M, et al. Comparison of five in vitro digestion models to study the bioaccessibility of soil contaminants. Environ Sci Technol 2002;36:3326-34.
80. Frederiksen M, Vorkamp K, Thomsen M, Knudsen LE. Human internal and external exposure to PBDEs - a review of levels and sources. Int J Hyg Environ Health 2009;212:109-34.
81. Rahman M. E-waste management challenges in the country. 2021. Available from: https://today.thefinancialexpress.com.bd/views-opinion/e-waste-management-challenges-in-the-country-1618664674. [Last accessed on 11 Dec 2024].
Cite This Article
How to Cite
Download Citation
Export Citation File:
Type of Import
Tips on Downloading Citation
Citation Manager File Format
Type of Import
Direct Import: When the Direct Import option is selected (the default state), a dialogue box will give you the option to Save or Open the downloaded citation data. Choosing Open will either launch your citation manager or give you a choice of applications with which to use the metadata. The Save option saves the file locally for later use.
Indirect Import: When the Indirect Import option is selected, the metadata is displayed and may be copied and pasted as needed.
About This Article
Special Issue
Copyright
Data & Comments
Data
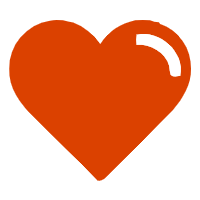
Comments
Comments must be written in English. Spam, offensive content, impersonation, and private information will not be permitted. If any comment is reported and identified as inappropriate content by OAE staff, the comment will be removed without notice. If you have any queries or need any help, please contact us at support@oaepublish.com.