Effects of early life exposure to di(2-ethylhexyl) phthalate on jejunal morphology, sucrase activity, and colonic microbiota composition in young pigs
Abstract
The diesters of 1,2-benzenedicarboxylic acid (phthalic acid), or phthalates, are multifunctional chemicals used in personal care products, medications, and plastics. Phthalate metabolites are also found in human milk and infant formula. However, their impact on intestinal development and microbiota composition in early life is understudied. Herein, the effect of di-(2-ethylhexyl) phthalate (DEHP) on growth, intestinal morphology, enzyme activity, and microbiota composition was assessed. Piglets (two-day-old, n = 24) were randomized to receive either 20 mg (DEHP20) or 200 mg (DEHP200)/kg body weight (BW)/day in corn oil. A control group (CON, 0 mg phthalate) received the same volume of corn oil as DEHP200. After 21 days, tissue and urine samples were collected. DEHP did not affect weight gain. Urinary DEHP metabolite concentrations increased in a dose-dependent fashion. Jejunal villus length was significantly shorter in the DEHP200 than CON and DEHP20, while villus area was smaller in DEHP200 than DEHP20 but not CON. Crypt depth and area were higher in DEHP200 than DEHP20, but neither differed from CON. Additionally, jejunal sucrase activity was higher in the DEHP200 than CON. Bacterial alpha diversity differed significantly between DEHP groups and CON in the ascending colon, while beta diversity revealed significant differences between DEHP200 and CON and DEHP20. The abundance of Christensenellaceae R-7 group, Romboutsia, Lachnospiraceae UCG-004, Odoribacter, and Sphaerochaeta, among others, differed in DEHP-exposed vs. CON animals. Thus, daily exposure to phthalates during infancy changes the villus structure and disaccharidase activity in the small intestine and these changes may be modulated by the colonic bacterial community.
Keywords
INTRODUCTION
Widespread exposure to environmental contaminants poses a significant concern for human health. Epidemiological studies have reported associations between health outcomes and exposure to phthalates, a ubiquitous plasticizer that gives plastics characteristic transparency and flexibility and is commonly utilized in food packaging, personal care products, and medical devices[1]. Phthalate exposure occurs through ingestion, inhalation, dermal, and parenteral routes and has been linked to endocrine and reproductive disruptions in humans and animals[2,3].
The time between conception and the first two years of life is critical for human development and is likely to be the most sensitive period of the lifespan to environmental exposures[4]. Indeed, epidemiological studies have shown that pre- and postnatal exposure to environmental contaminants, including phthalates, are associated with alterations in infant and toddler physical and behavioral development[5]. In addition, metabolic abnormalities, such as increased body weight (BW), atypical adipogenesis, energy expenditure, and glucose metabolism, are observed in mice exposed to di-(2-ethylhexyl) phthalate (DEHP) prenatally or in adulthood[6,7]. Additionally, there is concern regarding the potential accumulation of toxicant concentrations and an extended excretion period in infants during their early life stages due in part to their immature capacity to detoxify environmental contaminants[8,9].
The primary sources of nutrition for infants in the first 6 months of life, human milk and infant formula, are sources of environmental contaminants; phthalate metabolites are present in both human milk and formula[10-12]. A recent study in Southern China found that breastfed infants had an estimated phthalate intake between 0.383 to 6.95 µg/kg BW[10], which is below the tolerable daily intake of 50 µg/kg BW set by the European Food Safety Authority[13]. Infants and children are frequently exposed to other sources of plasticizers present in bottles, toys, lotions, and, in some instances, medical devices. While some human studies have reported detrimental developmental impacts of phthalate exposure during the early postnatal period, a recent systematic review has concluded that quantitative evidence on the effects of exposure to phthalates during this period is limited and studies have methodological limitations[14]. Additionally, most data on early-life phthalate exposure are derived from rodent studies.
The present study aimed to assess the phthalate-mediated early-life impact on the gastrointestinal tract in the neonatal pig, a well-characterized biomedical model for human infants. The neonatal piglet has been used extensively to understand the influence of environmental factors in the early developmental stages of life due to its shared similarities in gastrointestinal anatomy and a closer similarity in gut microbiota composition to humans than any other species[15,16]. Furthermore, the neonatal pig is a model for assessing the interactions between microbiota and health, as it exhibits disorders similar to those in humans[16]. The DEHP dosages were selected based on previous work from our group, which has been shown to have long-term detrimental effects on female reproductive health in mice[17,18]. We hypothesized that exposure to DEHP in milk replacer formula would alter intestinal morphology, digestive enzyme activity, and microbiota composition relative to animals not exposed to DEHP.
EXPERIMENTAL
Animal care and housing
The study design and animal protocol were described by Lee et al.[19]. Briefly, two-day-old piglets (n = 24) (12 males and 12 females from four different litters) were obtained from the Swine Research Center at the University of Illinois in Urbana-Champaign and transferred to the AAALAC-approved animal facilities in the Edward R. Madigan Laboratory on the University of Illinois in Urbana-Champaign campus. Upon arrival at the animal facility, piglets were randomized by initial BW and sex to three dietary treatments, where there were eight piglets (four females and four males in each treatment group). All animal and experimental procedures were in accordance with the National Research Council Guide for the Care and Use of Laboratory Animals[20] and approved by the University of Illinois in Urbana-Champaign Institutional Animal Care and Use Committee (protocol # 18112).
Phthalate exposures
Piglets were assigned to receive DEHP at dose levels of 0 (CON), 20 (DEHP20), or 200 (DEHP200) mg/kg BW/day. The DEHP (Sigma-Aldrich, St. Louis, MO) was dissolved in tocopherol-stripped corn oil and added to the feeding bowl prior to the first feeding of the day from postnatal day (PND) 3-23. The CON group received a daily dose of DEHP-free tocopherol-stripped corn oil at the volume of the DEHP200. The DEHP doses reflect those previously reported in the literature[21-23]. The 20 mg/kg/day dose aligns with exposure levels from medical devices[21,22]. The 200 mg/kg/day dose of DEHP has been used in previous studies by our group[23]. Piglets were fed a nutritionally adequate sow-milk replacer formula reconstituted at 18.3% w/v (Milk Specialties Global, Eden Prairie, Minnesota). Piglet BW was measured daily, and the volume of the formula provided was based on 300 mL/kg BW/day (PND3-7) and 325 mL/kg BW/day (PND8-23). Formula intake was calculated by subtracting daily formula waste from the formula provided.
Sample collection
On PND23, animals were sedated with an intramuscular injection of Telazol® (Tiletamine HCl and Zolazepam HCl, 3.5 mg/kg BW each, Pfizer Animal Health, Fort Dodge, IA) followed by an intravenous injection of 86 mg/kg BW sodium pentobarbital (Euthasol; Virbac AH, Inc., Fort Worth, TX). The spleen, brain, kidneys, heart, lungs, intestine, and liver were excised and weighed. Samples from the small and large intestines were fixed in 10% buffered formalin for morphological analysis. The small intestine mucosa was scraped using a microscope slide and snapped-frozen to assess disaccharidase activity. Ascending colon (AC) and rectal contents (RC) were collected for microbiota analysis. In addition, urine samples were collected via cystocentesis (urine collected directed from the bladder using a sterile needle and syringe) for urinary DEHP metabolites assessment by liquid chromatography/mass spectrometry (LC/MS/MS).
Histomorphological analyses
Formalin-fixed sections of duodenum, jejunum, ileum, and AC were processed into paraffin blocks, sectioned into 5-μm slices, and placed on glass slides. Digital images (10× magnification) of hematoxylin-eosin–stained tissues were captured with the use of the NanoZoomer Slider Scanner (Hamamatsu Corporation; Institute for Genomic Biology Core Microscopy and Imaging Facility, UI). Small intestinal villus height (μm), crypt depth (μm), and colon cuff depth (μm), and villus, crypt, and colon cuff areas (mm2) were measured from 10-15 well-oriented crypts-villi with AxioVision 4.8 Digital Image Processing Software (Carl Zeiss MicroImaging, Inc.).
Disaccharidase activity
Intestinal lactase and sucrase activities were measured as previously described[24,25]. Small intestine mucosal homogenates were prepared and incubated with 0.6 M lactose (ThermoFisher Scientific, Waltham, MA) or 0.3 M sucrose (ThermoFisher Scientific) buffer (dissolved in 0.0625 M maleate buffer) for 60 min at 37 °C. The reaction was stopped by the addition of 2.0% zinc sulfate (ThermoFisher Scientific) and 1.8% barium hydroxide (ThermoFisher Scientific), and the amount of glucose released was detected with a glucose oxidase reagent (ThermoFisher Scientific). The Bradford method measured protein in intestinal homogenates (Bio-Rad Laboratories, Inc, Hercules, CA). Enzyme activity was expressed as μmoles of glucose per minute per gram of protein.
Urinary DEHP metabolites
Urinary DEHP metabolites were assessed as described by Warner et al.[26]. Mono-(2-ethyl-5-hydroxyhexyl) phthalate (MEHHP), mono-(2-ethyl-5-hexyl) phthalate (MEHP), mono-(2-ethyl-5-oxohexyl) phthalate (MEOHP) and mono-(2-ethyl-5-carboxypentyl) phthalate (MECPP) were analyzed in the 5500 QTRAP LC/MS/MS system (Sciex, Framingham, Massachusetts) at the Metabolomics Lab of the Roy J. Carver Biotechnology Center (UIUC) and Analyst 1.6.2 software was used for data acquisition.
Statistical analyses
Analysis of variance (ANOVA) was conducted using the MIXED procedure of SAS 9.4 (SAS Institute Inc., Cary, NC, USA) to differentiate dose effects on young pigs. Depending on the outcome, one of two statistical models was used: (1) data collected at a single time point were analyzed by 1-way ANOVA; and (2) data collected from the same animal on more than one occasion (i.e., daily BW and formula intake) were analyzed by ANOVA with repeated measures, in which dietary treatment, time, and treatment by time interaction were added to the statistical model. Normality was checked by the Shapiro-Wilk test and data were log-transformed if distribution did not follow normal distribution (urinary metabolite data). Outliers were identified by a studentized residual procedure where values of > 3 or < -3 were removed from the statistical analysis. Data were expressed as mean ± standard error of the means (SEM) and statistical significance was defined at P < 0.05. Microbial statistical analyses are described below.
Microbiota analysis
DNA extraction
DNA was extracted from AC and RC contents with the QIAamp DNA Stool Mini Kit (Qiagen, Germantown, MD) in combination with the FastPrep-24 System (MP Biomedicals, Solon, OH) as previously described[27]. DNA concentration was determined with a NanoDrop 1000 spectrophotometer (NanoDrop Technologies, Wilmington, DE).
Polymerase chain reaction amplification, library preparation, and sequencing of 16S rRNA genes
Polymerase chain reaction (PCR) amplification, library preparation, and sequencing of 16S rRNA genes were performed at Roy J. Carver Biotechnology Center, UIUC. The full-length 16S rRNA genes were amplified from the extracted DNA using universal primers 27F: AGRGTTYGATYMTGGCTCAG and 1492R: RGYTACCTTGTTACGACTT[28]. For each sample, both the forward and reverse primers were tailed with sample-specific PacBio barcode sequences to allow for multiplexed sequencing. The PCR amplification mixture contained 0.3 µM of both primers, 2.5 ng of genomic DNA, 12.5 µL Kappa Hotstart Ready Mix (Roche Sequencing and Life Science, Wilmington, MA) in a final volume of 25 µL. PCR temperature profile included 1 cycle at 95 °C for 3 min, followed by 25 cycles at 95 °C for 30 s, 57 °C for 30 s, and 72 °C for
Sequence processing
Sequences were processed using DADA2 R package and QIIME 2 pipeline[29,30]. Demultiplexed reads obtained from the sequencing facility were subjected to primer removal, dereplication, denoising, and chimera checking using DADA2 package of R (version 1.14.1)[29]. The amplicon sequence variant (ASV) table and representative sequences generated from DADA2 were imported and further analyzed in QIIME pipeline (version 2020.6)[30]. The representative sequences were aligned, and a phylogenetic tree was constructed from filtered alignment as previously[31]. Alpha diversity and UniFrac distance metrics were computed through the plugin diversity with the core metrics-phylogenetic method on the ASV table and phylogenetic tree. Samples were rarefied to an equal number of reads (10,956) when α-diversity and UniFrac distance metrics were calculated. Taxonomic assignments were performed using plugin feature-classifier with classify-sklearn method[32,33], in which a pre-trained Naïve-Bayes classifier with SILVA 138 99% operational taxonomic units (OTUs) full-length sequences was used (https://www.arb-silva.de/)[34]. Taxonomic bar plots were generated using the plugin taxa with the taxa barplot method. The bar plots were visualized, and the count tables at the phylum and genus levels were downloaded using QIMME 2 View
Microbiota statistical analyses
Differences in overall bacterial communities among treatment groups (beta-diversity) were evaluated with principal coordinate analysis (PCoA) and permutational multivariate analysis of variance (PERMANOVA). PERMANOVA was performed on weighted UniFrac distances in QIIME 2 using plugin diversity with beta-group-significance method[30]. Alpha diversity [Shannon’s indices, Evenness, Faith’s phylogenic diversity (PD)] was analyzed using the PROC MIXED procedure of SAS (version 9.4; SAS Institute). For differential abundance analysis, counts of bacterial taxa were assessed with pairwise comparisons using a negative binomial Wald test implemented in the R package DESeq2[35]. Genera with mean relative abundance < 0.01% and present in < 20% of samples were removed from the differential abundance analysis. Data are reported as means ± SEM or log2 fold change. Statistical significance was defined as P ≤ 0.05 for alpha and beta diversity analysis. For differential abundance of bacterial taxa, P-values were adjusted (Padj) for multiple testing by the Benjamini–Hochberg procedure, and a Padj ≤ 0.10 was considered statistically significant.
RESULTS
Growth, formula intake, and organ weights
There were no differences in BW over the course of the study between the piglets dosed with 20 or 200 mg DEHP/kg BW/day compared to CON [Figure 1]. Total BW gain, calculated as the difference in weights between PND23 and PND2, did not differ among the groups, and the gains were 6.2 ± 0.07, 6.2 ± 0.1 and 6.0 ±
Figure 1. BW of piglets fed formula containing 0 (CON), 20 (DEHP20) or 200 (DEHP200) mg DEHP/kg BW/day from postnatal days 2 to 23. Values are means ± SEM. There were no significant differences in weight gain (P > 0.05). DEHP: Di-(2-ethylhexyl) phthalate; BW: body weight; SEM: standard error of the means.
Small and large intestinal weight and length and organ weights normalized by BW of piglets supplemented orally with 0 (CON), 20 (DEHP20), or 200 (DEHP200) mg DEHP/kg BW/day
Treatment groups | P-value | |||
CON | DEHP20 | DEHP200 | ||
Intestinal length (cm/kg) | 116 ± 2.5 | 118 ± 2.6 | 116 ± 2.0 | 0.74 |
Intestinal weight (g/kg) | 34 ± 0.9 | 34 ± 1.2 | 34 ± 1.4 | 0.98 |
Cecum + colon (g/kg) | 23 ± 1.1 | 22 ± 0.9 | 22 ± 0.7 | 0.53 |
Brain (g/kg) | 6.2 ± 0.1 | 6.0 ± 0.1 | 6.3 ± 0.2 | 0.34 |
Kidneys (g/kg) | 7.5 ± 0.3 | 7.7 ± 0.3 | 8.0 ± 0.5 | 0.59 |
Spleen (g/kg) | 3.5 ± 0.5 | 4.2 ± 0.7 | 3.5 ± 0.6 | 0.68 |
Heart (g/kg) | 6.1 ± 0.2 | 6.0 ± 0.2 | 6.7 ± 0.3 | 0.06 |
Liver (g/kg) | 33 ± 0.9 | 31 ± 0.7 | 32 ± 0.7 | 0.20 |
Lung (g/kg) | 13 ± 0.3 | 13 ± 0.5 | 13 ± 0.5 | 0.63 |
Urinary metabolites
Four different DEHP metabolites were detected in urine [Figure 2]. DEHP metabolite excretion in the urine was positively correlated with DEHP dose. MEHP, MEHHP, MEOHP, and MECPP concentrations in DEHP20 and DEHP200 were 6.6-, 7.5-, 6.3-, and 6.3-fold, and 51-, 115-, 47-, and 38-fold higher than CON, respectively, while DEHP200 was 7.7-, 15.2-, 7.6-, and 6.1-fold higher than DEHP-20. MEOHP was the most abundant DEHP metabolite found in urine, followed by MEHHP and MEHP.
Figure 2. Urinary metabolite concentration in piglets administered 0 (CON), 20 (DEHP20) or 200 (DEHP200) mg DEHP/kg BW/day on PND23. Values are means ± SEM. Different letters superscripts for each metabolite indicate statistical differences at P < 0.0001. n = 8 per group. MEHHP: Mono-(2-ethyl-5-hydroxyhexyl) phthalate; MEHP: mono-(2-ethyl-5-hexyl) phthalate; MEOHP: mono-(2-ethyl-5-oxohexyl) phthalate; MECPP: mono-(2-ethyl-5-carboxypentyl) phthalate; DEHP: di-(2-ethylhexyl) phthalate; BW: body weight; SEM: standard error of the means.
Intestinal histomorphology
Morphological measurements of small intestine villi and crypts and AC cuffs are presented in Table 2. Exposure to DEHP did not affect intestinal morphology in the ileum or AC. A statistical trend (P < 0.07) was observed toward higher crypt depth in the duodenum of DEHP200 compared to CON and DEHP20. Jejunal villus length was significantly shorter in piglets fed the high dose of DEHP, while villus area was lower in DEHP200 than DEHP20. Jejunum villus area between DEHP and CON tended (P = 0.07) to differ. Jejunal crypt depth was highest in DEHP200, intermediate in CON, and lowest in DEHP20, while crypt area was lower in DEHP20 than CON and DEHP200.
Small and large intestine morphology of piglets supplemented orally with 0 (CON), 20 (DEHP20), or 200 (DEHP200) mg DEHP/kg BW
Treatment groups | P-value | |||
CON | DEHP20 | DEHP200 | ||
Duodenum | ||||
Villus length (μm) | 547 ± 36 | 543 ± 25 | 594 ± 28 | 0.42 |
Villus area (mm2) | 67.0 ± 5.2 | 69.1 ± 4.3 | 74.8 ± 6.0 | 0.56 |
Crypt depth (μm) | 250 ± 5.0 | 245 ± 10 | 276 ± 12 | 0.07 |
Crypt area (mm2) | 12.6 ± 0.9 | 11.5 ± 0.7 | 13.1 ± 0.9 | 0.39 |
Jejunum | ||||
Villus length (μm) | 557 ± 16a | 569 ± 22a | 476 ± 26b | 0.013 |
Villus area (mm2) | 63.8 ± 2.8a,b,* | 70.1 ± 4.4a | 52.2 ± 5.0b | 0.019 |
Crypt depth (μm) | 160 ± 5.0a,b | 143 ± 7.9a | 170 ± 7.4b | 0.039 |
Crypt area (mm2) | 6.5 ± 0.3a | 5.2 ± 0.4b | 6.8 ± 0.6a | 0.027 |
Ileum | ||||
Villus length (μm) | 435 ± 21 | 427 ± 10 | 415 ± 16 | 0.70 |
Villus area (mm2) | 46.6 ± 3.8 | 44.8 ± 1.8 | 43.4 ± 1.9 | 0.71 |
Crypt depth (μm) | 179 ± 7.5 | 172 ± 5.7 | 169 ± 3.4 | 0.50 |
Crypt area (mm2) | 6.7 ± 0.3 | 6.3 ± 0.1 | 6.5 ± 0.2 | 0.31 |
Ascending colon | ||||
Crypt depth (μm) | 285 ± 15 | 262 ± 8.3 | 256 ± 6.7 | 0.15 |
Crypt area (mm2) | 15.7 ± 1.2 | 13.3 ± 0.8 | 14.1 ± 0.6 | 0.18 |
Intestinal lactase and sucrase activities
Disaccharidase activities were assessed in the duodenum, jejunum, and ileum [Table 3]. Oral DEHP had no impact on intestinal lactase activity or sucrase activity in the duodenum or ileum. Sucrase increased in a dose-dependent fashion in the jejunum, with the highest activity in the DEHP200, intermediary in the DEHP20, and lowest in CON.
Small intestine disaccharidase activity (μmol/min/g protein) of piglets supplemented orally with 0 (CON), 20 (DEHP20), or 200 (DEHP200) mg DEHP/kg BW
Treatment groups | P-value | |||
CON | DEHP20 | DEHP200 | ||
Duodenum | ||||
Sucrase activity | 23.9 ± 4.9 | 31.7 ± 5.4 | 43.3 ± 7.8 | 0.12 |
Lactase activity | 72.7 ± 13.4 | 103 ± 13.0 | 114 ± 20.1 | 0.28 |
Jejunum | ||||
Sucrase activity | 95.4 ± 8.5a | 159 ± 11.8b | 209 ± 22.7c | 0.0002 |
Lactase activity | 129 ± 10.1 | 107 ± 26.8 | 147 ± 22.6 | 0.42 |
Ileum | ||||
Sucrase activity | 77.4 ± 14.3 | 116 ± 23.4 | 77.5 ± 8.8 | 0.19 |
Lactase activity | 49.5 ± 16.8 | 41.6 ± 6.4 | 39.0 ± 10.0 | 0.81 |
Intestinal microbiota
Alpha diversity is a measure of the diversity of ecological communities, which can be described using indices such as richness, evenness, the Shannon index, and Faith’s PD. The Shannon index considers both species richness (how many different species are present) and evenness (how evenly species are distributed). Faith’s PD measures the diversity of a microbial community by considering the phylogenetic relationships between species, rather than just their presence or abundance[36]. DEHP exposure notably enhanced alpha diversity in the AC contents compared to CON, as evidenced by the increased number of observed features and Shannon index [Table 4]. In the RC, although the number of observed features tended to be higher in the DEHP200 group relative to CON (P = 0.073), other indices did not show significant differences between the groups. Examination of overall bacterial communities through PCoA and PERMANOVA indicated that bacterial communities were similar between CON and DEHP20 (P > 0.05). However, both CON and DEHP20 significantly differed from DEHP200 in both AC and RC [Figure 3].
Alpha diversity measures of ascending colon and rectal contents of piglets supplemented orally with 0 (CON), 20 (DEHP20), or 200 (DEHP200) mg DEHP/kg BW/day
Treatment groups | P-value | |||
CON | DEHP20 | DEHP200 | ||
Ascending colon | ||||
Observed features | 365 ± 24.8a | 451 ± 21.8b | 448 ± 18.2b | 0.012 |
Evenness | 0.7 ± 0.01 | 0.73 ± 0.01 | 0.74 ± 0.01 | 0.073 |
Shannon index | 5.9 ± 0.17a | 6.4 ± 0.09b | 6.5 ± 0.14b | 0.0117 |
Faith’s PD | 16.3 ± 0.55 | 17.6 ± 0.58 | 17.1 ± 0.4 | 0.19 |
Rectum | ||||
Observed features | 392 ± 18.5 | 421 ± 23 | 462 ± 22.9 | 0.069 |
Evenness | 0.74 ± 0.02 | 0.74 ± 0.01 | 0.73 ± 0.01 | 0.99 |
Shannon index | 6.4 ± 0.23 | 6.4 ± 0.14 | 6.5 ± 0.16 | 0.83 |
Faith’s PD | 17.9 ± 0.51 | 18.7 ± 0.75 | 18.5 ± 0.58 | 0.64 |
Figure 3. Principal co-ordinate analysis based on weighted UniFrac distances generated from ascending colon (A) and rectal (B) contents of piglets administered with 0 (CON), 20 (DEHP20) or 200 (DEHP200) mg DEHP/kg BW/day. DEHP: Di-(2-ethylhexyl) phthalate; BW: body weight.
Analysis of differential abundance revealed significant alterations in bacterial composition due to DEHP exposure at both phyla and genera levels. At the phyla level, no differences were observed in bacterial abundances between the CON and DEHP20 in either the AC or RC contents [Table 5]. The AC contents of piglets fed DEHP200 exhibited increased levels of Actinobacteria, Bacillota (formerly known as Firmicutes), and Verrucomicrobiota compared to CON. Conversely, AC contents of piglets fed DEHP200 showed decreased abundances of Bacteroidota and Spirochaetota relative to both CON and DEHP20. In the RC, bacterial abundances of piglets fed DEHP200 did not differ significantly from CON piglets. However, RC contents of DEHP200 piglets had higher relative abundances of Actinobacteria and Bacillota, while Bacteroidota were lower than DEHP20 [Table 5].
Bacterial phyla that significantly differed in the ascending colon and rectal contents of piglets supplemented orally with 0 (CON), 20 (DEHP20), or 200 (DEHP200) mg DEHP/kg BW/day
Bacterial phyla | Base mean | DEHP20/CON | DEHP200/CON | DEHP200/DEHP20 | |||
LFC | Padj | LFC | Padj | LFC | Padj | ||
Ascending colon | |||||||
Actinobacteriota | 720.3 | 1.85 | 0.355 | 2.44 | 0.024 | 0.59 | 0.787 |
Bacteroidota | 11,705.8 | -0.02 | 0.965 | -1.07 | 0.012 | -1.06 | 0.014 |
Bacillota | 10,727.8 | 0.32 | 0.471 | 0.67 | 0.012 | 0.35 | 0.358 |
Spirochaetota | 191.2 | 1.60 | 0.693 | -25.12 | < 0.001 | -26.72 | < 0.001 |
Verrucomicrobiota | 50.3 | 1.33 | 0.525 | 2.54 | 0.050 | 1.20 | 0.627 |
Rectum | |||||||
Actinobacteriota | 670.0 | -0.10 | 0.95733 | 1.60 | 0.115 | 1.70 | 0.092 |
Bacteroidota | 9,352.1 | 0.54 | 0.81531 | -0.95 | 0.115 | -1.50 | 0.006 |
Bacillota | 18,693.0 | -0.09 | 0.95733 | 0.70 | 0.115 | 0.78 | 0.092 |
Table 6 illustrates alterations in AC bacterial genera. Exposure to phthalates at either dose increased the abundance of Christensenellaceae R-7 group and Romboutsia and decreased the relative abundance of Lachnospiraceae UCG-014 compared to the CON group. Additionally, DEPH200 animals displayed lower levels of Odoribacter, Sphaerochaeta, Clostridia UCG-014, [Eubacterium] Fissicatena, and Rominococcus compared to both CON and DEHP20. The abundance of bacteria from the genera Lactobacillus and Clostridium sensu stricto 1 was higher in the AC contents of the DEHP200 group than the CON but not the DEHP20 group. Lastly, exposure to DEHP20 led to a significant decrease in the relative abundance of Holdemanella and Clostridia Vadim BB60 compared to both CON and DEHP200.
Bacterial genera that significantly differed in the ascending colon contents of piglets supplemented orally with 0 (CON), 20 (DEHP20), or 200 (DEHP200) mg DEHP/kg BW/day
Bacterial genus | Base mean | DEHP20/CON | DEHP200/CON | DEHP200/DEHP20 | |||
LFC | Padj | LFC | Padj | LFC | Padj | ||
Bacteroidota | |||||||
Bacteroides | 7,778.4 | -1.07 | 0.458 | -1.82 | 0.020 | -0.75 | 0.591 |
Odoribacter | 197.2 | -0.90 | 0.958 | -26.7 | < 0.001 | -25.8 | < 0.001 |
Sanguibacteroides | 39.3 | -1.48 | 0.852 | -5.67 | 0.024 | -4.19 | 0.250 |
Spirochaetota | 191.2 | 1.60 | 0.693 | -25.12 | < 0.001 | -26.72 | < 0.001 |
Verrucomicrobiota | 50.3 | 1.33 | 0.525 | 2.54 | 0.050 | 1.20 | 0.627 |
Bacillota | |||||||
Holdemanella | 376.0 | -6.36 | 0.011 | 0.94 | 0.803 | 7.30 | 0.001 |
Lactobacillus | 702.0 | 0.71 | 0.693 | 1.44 | 0.098 | 0.73 | 0.591 |
RF39 | 32.0 | 2.33 | 0.693 | -3.46 | 0.277 | -5.79 | 0.024 |
Christensenellaceae R-7 | 264.1 | 1.92 | 0.011 | 2.00 | 0.003 | 0.08 | 0.945 |
Clostridia UCG-014 | 188.9 | -0.62 | 0.693 | -2.14 | 0.002 | -1.52 | 0.046 |
Clostridia vadin BB60 | 49.6 | -4.68 | 0.011 | -3.03 | 0.141 | 1.65 | 0.591 |
Clostridium sensu stricto 1 | 102.6 | 1.44 | 0.693 | 3.00 | 0.059 | 1.56 | 0.591 |
[Eubacterium] fissicatena | 203.8 | -0.66 | 0.925 | -3.75 | 0.008 | -3.10 | 0.046 |
Lachnospiraceae UCG-004 | 30.9 | -4.73 | 0.084 | -6.06 | 0.006 | -1.33 | 0.786 |
Colidextribacter | 116.7 | 2.56 | 0.086 | 1.56 | 0.337 | -1.00 | 0.691 |
Ruminococcus | 212.2 | -0.12 | 0.958 | -1.98 | 0.024 | -1.86 | 0.046 |
Intestinibacter | 2.7 | -2.66 | 0.600 | -4.79 | 0.043 | -2.13 | 0.596 |
Romboutsia | 62.2 | 2.62 | 0.086 | 3.19 | 0.012 | 0.58 | 0.788 |
Spirochaetota | |||||||
Sphaerochaeta | 203 | 0.30 | 0.970 | -26.3 | < 0.001 | -26.6 | < 0.001 |
The influence of phthalates on RC revealed a significant decrease in the relative abundance of [Eubacterium] Fissicatena and Anaerovoracaceae Family XIII AD3011 in DEHP200 compared to CON alone [Table 7]. Meanwhile, the reduction in Sanguibacteroides was relative to both CON and DEHP20. Holdemanella levels decreased in DEHP20 compared to CON and DEHP200. In the pairwise comparison between DEHP200 and DEHP20, the genera [Clostridium] innocuum, Turicibacter, Leuconostoc, Lactococcus, Streptococcus, and Eubacterium were elevated, while Odoribacter, Alistipes, RF29, and Anaerovorax were reduced.
Bacterial genera that significantly differed in the rectal contents of piglets supplemented orally with 0 (CON), 20 (DEHP20), or 200 (DEHP200) mg DEHP/kg BW/day
Bacterial genus | Base mean | DEHP20/CON | DEHP200/CON | DEHP200/DEHP20 | |||
LFC | Padj | LFC | Padj | LFC | Padj | ||
Bacteroidota | |||||||
Odoribacter | 589.2 | 1.00 | 0.945 | -6.23 | 0.175 | -7.23 | 0.063 |
Sanguibacteroides | 97.0 | -0.39 | 0.952 | -3.84 | 0.107 | -3.45 | 0.084 |
Alistipes | 676.0 | 1.64 | 0.525 | -1.48 | 0.469 | -3.12 | 0.063 |
Bacillota | |||||||
[Clostridium] innocuum | 5.5 | -0.17 | 0.985 | 3.01 | 0.175 | 3.18 | 0.077 |
Holdemanella | 589.8 | -6.78 | 0.004 | 2.80 | 0.399 | 9.58 | < 0.001 |
Turicibacter | 153.8 | -0.67 | 0.934 | 1.99 | 0.322 | 2.66 | 0.085 |
Leuconostoc | 3.1 | -4.06 | 0.281 | 0.59 | 0.813 | 4.65 | 0.085 |
Lactococcus | 6.5 | -3.20 | 0.281 | 0.60 | 0.792 | 3.79 | 0.077 |
Streptococcus | 218.6 | -2.08 | 0.281 | 0.51 | 0.779 | 2.60 | 0.063 |
RF39 | 240.0 | 1.28 | 0.934 | -4.09 | 0.175 | -5.36 | 0.063 |
Eubacterium | 50.6 | -0.38 | 0.945 | 2.44 | 0.175 | 2.82 | 0.063 |
[Eubacterium] fissicatena | 428.4 | -0.77 | 0.720 | -2.11 | 0.107 | -1.35 | 0.266 |
Colidextribacter | 65.4 | 2.63 | 0.135 | 2.77 | 0.107 | 0.14 | 0.925 |
Anaerovorax | 9.8 | 0.39 | 0.945 | -2.28 | 0.175 | -2.67 | 0.063 |
Family XIII AD3011 | 56.6 | -0.97 | 0.525 | -2.19 | 0.026 | -1.21 | 0.210 |
DISCUSSION
This study aimed to investigate whether early postnatal exposure to DEHP results in deviations in intestinal development and function, as well as alterations in gut microbiota, compared to no exposure in neonatal pigs. The DEHP doses were in the range used in studies in rodents[37-39] and swine[40,41] or described to be the estimated exposure of infants in a neonatal intensive care unit[42]. We observed no effects on whole BW gain or organ growth, whereas effects on intestinal villus structure, disaccharidase activity, and colonic microbiota were observed.
Based on urinary metabolite profiles, neonatal pigs appear to metabolize DEHP similarly to newborn infants with respiratory distress who received IV fluid treatment[43]. Specifically, MEHHP, MEHP, MEOHP, and MECCP concentrations in DEHP-exposed piglets were significantly higher than controls and increased in a dose-dependent fashion. Diesters of phthalate metabolites are degraded by esterase and lipase to form monoesters that may further be transformed into oxidative metabolites in the intestine or other tissues[44]. Urinary metabolites are used as indicators of phthalates exposure and are used for biomonitoring of exposure. Although infants may not metabolize or eliminate phthalate byproducts as effectively as adults in the first 6 months of life, this capacity evolves over time[9].
In contrast to findings in rodents[7,45], exposure to DEHP at 20 or 200 mg/kg did not affect BW gain or organ size in pigs. However, significant changes in intestinal morphology and function were observed. The highest DEHP dose was associated with shorter villi but deeper crypts in the jejunum compared to CON, indicating villus atrophy and crypt hyperplasia. Comparable outcomes were noted in adult mice receiving a high dose (500 mg/kg BW) of dibutyl phthalate (DBP)[46]. In this investigation, the DBP-treated group had significantly reduced jejunal villus height and villus/crypt ratio compared to the control group, with no notable changes in crypt depth. Conversely, DBP administration had the opposite effect in the duodenum, leading to a significant increase in villus height and villus/crypt ratio[47]. Likewise, a study of quails exposed to high levels of DEHP (250, 500, 750 mg/kg BW) reported decreased villi length and increased crypt depth in the duodenum. As a result of the exposure, disruption of the epithelial barrier and varying degrees of intestinal inflammation were observed[47]. Diisononyl phthalate (DINP) fed to female rats during pregnancy and lactation caused severe villous atrophy in the 30-day-old offspring, while DEHP administration resulted in intestinal lymphoepithelial lesions[48]. The same study documented higher lactase and sucrase activities in the small intestine. Similarly, we noted a DEHP dose-dependent increase in sucrase activity in the jejunum, while no discernible impact of DEHP was observed in the duodenum and ileum. Beumer and Clevers[49] presented new insights into stem cell regulation and fate specification in response to intestinal insults or damage. They postulated that cells in the intestinal epithelium have a certain degree of plasticity that allows them to function as stem cells and display context-dependent functionality as dictated by their microenvironment. Others have hypothesized that the gut microbiota may modulate changes in intestinal function and structure after an insult such as DEHP exposure[50,51].
Many intestinal responses to environmental factors are associated with and modulated by the composition and diversity of the colonic microbiota. The establishment of the gut microbiota starts at birth and evolves during childhood until it reaches a composition that resembles those found in adults[52]. Microbial dysbiosis during early life has the potential to lead to chronic diseases, including inflammatory bowel disease, asthma, and obesity, among others[53]. In recent years, environmental chemicals have been shown to alter the microbiota. This study is the first, to our knowledge, to describe the significant effects of DEHP on the microbiota in young pigs. Others demonstrated that exposure to bisphenol A (BPA) at the concentration of 500 mg/kg BW/day (or 0.1% in the diet) resulted in impaired growth performance and intestinal morphology but observed no differences in alpha and beta diversity in young pigs[54]. In our study, bacterial richness (observed features and Shannon indices) increased significantly in the AC regardless of the DEHP dose, with no differences detected in the RC. Furthermore, differences in overall bacterial communities (beta diversity) separated the microbiota of animals from the high level of exposure from control and low level. Studies in pubertal female mice observed increased fecal alpha diversity (Chao1 index) after 7 days of oral administration of 1 or 10 mg DEHP/kg BW/day[55]. The effect disappeared after 14 days of treatment. In contrast, the work by Cheng et al. showed a significant decrease in microbial richness and structure in 6-week-old male C57BL/6 mice receiving 1,000 mg DEHP/kg BW/day for 15 weeks relative to non-exposed mice[56]. However, microbial diversity between control and animals exposed to 200 and 500 mg/kg BW/day did not differ.
To further explore the effect of DEHP on the composition of the microbiota, differential analyses at phylum and genus levels were performed. The distribution of bacteria at the phylum level did not differ between CON and DEHP20. DEHP200 led to significant changes in the abundance of Bacillota and Bacteroidota in the AC, which account for 90% of the sequences annotated in the colon content of young pigs[57]. The overall shift resulted in an increased abundance of Bacillota and Actinobacteria, accompanied by a decrease in Bacteroidota and Spirochaetota. Consistent with our findings, research involving 4-week-old female ICR mice administered 500 or 1,500 mg DEHP/kg BW/day[58], and pubertal Sprague-Dawley rats dosed with 3,000 mg DEHP/kg/BW/day[45] reported an increase in the ratio between Bacillota and Bacteroidota in fecal and cecal contents, respectively. The latter study found that DEHP doses of 300 and 1,000 mg/kg BW/day did not induce the same microbiota shift[45]. Differences in outcomes can be attributed to the use of different animal models and also, the dosage used, duration of exposure, and associated microbial changes[45].
To provide additional insight into the alterations in the microbiota, we performed differential analyses at the genus level. Animals exposed to any DEHP dose had a higher abundance of the Christensenellaceae R-7 and Romboutsia genera in the AC than CON. In the same animals, the abundance of Lachnospiraceae UCG-004 genus in DEPH20 and DEPH200 piglets was reduced compared to CON. Moreover, the AC contents of piglets exposed to DEHP200 exhibited lower abundances of Odoribacter and Sphaerochaeta compared to CON piglets. Interestingly, no difference was observed in either of these genera at the 20 mg DEHP/kg/day dose.
Christensenellaceae presence in the pig intestine is relatively low, with the colon harboring more of this bacterium than the ileum and cecum[59]. In humans, Christensenellaceae comprises approximately 0.01% of the fecal microbiota, and its relative abundance is affected by ethnicity, sex, age, and host genotype[60]. Christensenellaceae has emerged as an important modulator of health, and it is negatively associated with BMI, fat mass, serum lipids, blood pressure, and impaired glucose metabolism[60,61]. Furthermore, an increased abundance of species of the family Christensenellaceae has been strongly associated with asthma and asthma-associated intestinal metabolites in 3-year-old children[62]. However, no other study reported an increase in Christensenellaceae after exposure to phthalates. Romboutsia belongs to the Bacillota phylum. Its prevalence is elevated in various health conditions, such as neurodevelopmental disorders, immune-related chronic intestinal inflammation, and gastric cancer[63]. The higher abundance of Romboutsia observed at either DEHP dose in our study has also been reported in rodent studies[58,64,65].
Conversely, DEHP reduced the abundance of the genera Lachnospiraceae UCG-004 (Bacillota), Odoribacter (Bacillota), and Sphaerochaeta (Spirochaeota), all of which are known to metabolize polysaccharides and non-digestible fiber to produce volatile fatty acids (VFA)[66,67]. VFA are essential for gut integrity, provide an energy source for intestinal epithelial cells, and modulate the immune system[68]. In addition, VFA are important neuro-immuno-endocrine regulators and play significant roles in microbiota-gut-brain crosstalk like motility, secretion, and blood flow[69]. Fecal VFA were not measured in this study, but others reported that administration of high levels of DEHP significantly reduced fecal acetate and butyrate concentrations in rats[45]. The authors postulated that the DEHP-dependent change in the microbiota composition modulated the physiological effects of DEHP (severe organ damage, Th1 inflammatory response, and low fecal butyrate). However, these responses are rodent strain- and species-specific[45].
In the AC, the abundance of VFA-producing bacteria from the genera Holdomanella and Clostridia vadin BB60 group were lower in DEHP20 than CON, with no difference at the higher dose. A higher abundance of Holdemanella biformis was inversely correlated with irritable bowel symptoms following fecal microbial transplant[70]. Additionally, the AC contents of DEHP20 were enriched with Colidextribacter, a genus negatively correlated with BMI z-scores in 5-year-old children who had been born prematurely[71]. Others have reported that the effects of DEHP on the microbiota are not dose-dependent in rodents[45,58]. Herein, the overall microbiota structure in the AC and RC clustered more closely to CON than the DEHP200. We speculate that higher doses are needed to observe significant overall changes in the piglet model.
From a translational perspective, it is interesting to note that the effects of DEHP on the microbiota were more apparent in the AC contents compared to the RC contents, the latter of which is reflective of a fecal sample in humans. A higher number of phyla that differed in AC vs. RC (5 vs. 0) were observed between CON and DEHP200. Additionally, 6 and 13 bacterial genera differed between CON and DEHP20, and between CON and EDHP200, respectively, in AC, while only 1 and 4 differential genera were observed in RC between CON and DEHP20, and between CON and DEHP200, respectively. In this study, bacterial genera in the phyla Firmicutes were more affected by DEHP than those in the phylum Bacteroidota in samples from both the AC and RC. However, within the Bacteroidota, both Odoribacter and Sanguibacteroides were affected in AC and RC, whereas Bacteroides were also affected in the AC vs. Alistipes in the RC. Within the Bacillota, there were about an equal number of genera with differential abundance in response to DEHP. This should be considered when evaluating the impact of DEHP on the microbiome in human studies, as microbial dysbiosis in more proximal regions of the gut is likely underappreciated.
This study revealed for the first time notable alterations in small intestine structure and disaccharidase activity, as well as changes in colonic bacteria due to DEHP exposure, in the piglet model. However, several limitations warrant consideration. First, the doses administered exceeded typical DEHP exposure levels observed in healthy infants and children, although the DEHP20 dose reflected that found in the urine of pregnant women[72]. Second, we did not factor in potential secondary phthalate exposure from other dietary sources, plastic materials in the feeding system, or uncontrolled environmental factors. However, any additional exposure to phthalate contaminants affected all groups equally, minimizing potential compounding effects on the outcomes. Third, microbial taxonomy was assessed by 16S rRNA gene sequencing, and we did not measure fecal VFAs and other metabolites, which could have provided additional insights into the impact of microbiota functional changes. In addition, 16S rRNA gene sequencing does not capture the genetic differences that are known to exist in different strains of the same bacterium, as observed among the different strains of E. coli, with some being highly pathogenic while others are not. A recent study in mice[56] concluded that DEHP exposure causes an imbalance of intestinal microbial homeostasis, which results in a decrease in beneficial bacteria and an expansion of pathogenic bacteria abundance. The analyses of fecal metabolome indicated metabolic profiles were also changed due to DEHP and caused intestinal barrier dysfunction and activation of the AhR/NF-κB pathway to induce intestinal inflammation. Unfortunately, it is still unclear if the change in the metabolic profile was caused by DEHP or by dysbiosis[56]. Future studies could use gnotobiotic animals not directly exposed to DEHP but implanted with microbiota from DEHP-exposed animals. This approach would enable the determination of the specific effects of a DEHP-modified microbiota on host health. Future studies should use DEHP infant exposure levels and should incorporate metagenomic sequencing and metabolomic analyses to gain broader insight into the potential effects of DEHP on microbiome function.
CONCLUSION
This study documented that DEPH, a widely recognized endocrine-disrupting chemical, alters both the structure and function of the small intestine and induces changes in the colonic microbial community. The extent to which these microbiota structural changes contribute to alterations in intestinal development requires further study. Herein, we confirmed that the neonatal piglet is a suitable model for investigating how phthalate impacts development in early life. Building on previous findings[19], this study sheds light on the complex effects of phthalates, emphasizing the importance of continued inquiry into their biological ramifications. Furthermore, our findings highlight the potential health risks associated with DEHP exposure during critical periods of development. Future research should focus on the long-term consequences of early-life exposure to DEHP and other phthalates, including potential impacts on growth, immune function, and overall health. Additionally, exploring interventions to mitigate these effects could be valuable. Understanding the mechanisms underlying DEHP-induced changes in the gut microbiome and intestinal structure will be crucial for developing targeted strategies to protect vulnerable populations, such as infants, from the adverse effects of phthalate exposure.
DECLARATIONS
Authors’ contributions
Made substantial contributions to study conception and design: Donovan SM, Flaws JA, Irudayaraj JM, Cann I
Performed sample and data analysis and interpretation: Monaco MH, Coyne S, Wang M, Donovan SM
All authors approved the final manuscript.
Availability of data and materials
The datasets generated during the current study are available from the corresponding author upon reasonable request.
Financial support and sponsorship
Funding was provided by the Microbiome Metabolic Engineering Theme at the Carl R. Woese Institute for Genomic Biology, University of Illinois at Urbana-Champaign, Urbana, IL.
Conflicts of interest
All authors declared that there are no conflicts of interest.
Ethical approval and consent to participate
All animal and experimental procedures were approved by the Unversity of Illinois at Urbana-Champaign Institutional Animal Care and Use Committee (protocol # 18112).
Consent for publication
Not Applicable.
Copyright
© The Author(s) 2024.
Supplementary Materials
REFERENCES
1. Dueñas-Moreno J, Mora A, Kumar M, Meng XZ, Mahlknecht J. Worldwide risk assessment of phthalates and bisphenol A in humans: the need for updating guidelines. Environ Int 2023;181:108294.
2. Meeker JD, Ferguson KK. Urinary phthalate metabolites are associated with decreased serum testosterone in men, women, and children from NHANES 2011-2012. J Clin Endocrinol Metab 2014;99:4346-52.
3. Laws MJ, Neff AM, Brehm E, Warner GR, Flaws JA. Endocrine disrupting chemicals and reproductive disorders in women, men, and animal models. Adv Pharmacol 2021;92:151-90.
4. Heindel JJ. The developmental basis of disease: update on environmental exposures and animal models. Basic Clin Pharmacol Toxicol 2019;125 Suppl 3:5-13.
5. Braun JM, Sathyanarayana S, Hauser R. Phthalate exposure and children’s health. Curr Opin Pediatr 2013;25:247-54.
6. Fan Y, Qin Y, Chen M, et al. Prenatal low-dose DEHP exposure induces metabolic adaptation and obesity: role of hepatic thiamine metabolism. J Hazard Mater 2020;385:121534.
7. Su H, Yuan P, Lei H, et al. Long-term chronic exposure to di-(2-ethylhexyl)-phthalate induces obesity via disruption of host lipid metabolism and gut microbiota in mice. Chemosphere 2022;287:132414.
8. Martín-Carrasco I, Carbonero-Aguilar P, Dahiri B, Moreno IM, Hinojosa M. Comparison between pollutants found in breast milk and infant formula in the last decade: a review. Sci Total Environ 2023;875:162461.
9. Liu L, Wang H, Li X, et al. Infantile phthalate metabolism and toxico/pharmacokinetic implications within the first year of life. Environ Int 2020;144:106052.
10. Liu Y, Xiao M, Huang K, et al. Phthalate metabolites in breast milk from mothers in Southern China: occurrence, temporal trends, daily intake, and risk assessment. J Hazard Mater 2024;464:132895.
11. Krithivasan R, Miller GZ, Belliveau M, et al. Analysis of ortho-phthalates and other plasticizers in select organic and conventional foods in the United States. J Expo Sci Environ Epidemiol 2023;33:778-86.
12. Kim JH, Moon N, Ji E, Moon HB. Effects of postnatal exposure to phthalate, bisphenol a, triclosan, parabens, and per- and poly-fluoroalkyl substances on maternal postpartum depression and infant neurodevelopment: a korean mother-infant pair cohort study. Environ Sci Pollut Res Int 2023;30:96384-99.
13. Lambré C, Barat Baviera JM, Bolognesi C, et al; EFSA Panel on Food Contact Materials, Enzymes and Processing Aids (CEP). Identification and prioritisation for risk assessment of phthalates, structurally similar substances and replacement substances potentially used as plasticisers in materials and articles intended to come into contact with food. EFSA J 2022;20:e07231.
14. Laccetta G, Di Chiara M, Cardillo A, De Nardo MC, Terrin G. The effects of industrial chemicals bonded to plastic materials in newborns: a systematic review. Environ Res 2023;239:117298.
15. Guilloteau P, Zabielski R, Hammon HM, Metges CC. Nutritional programming of gastrointestinal tract development. Is the pig a good model for man? Nutr Res Rev 2010;23:4-22.
16. Heinritz SN, Mosenthin R, Weiss E. Use of pigs as a potential model for research into dietary modulation of the human gut microbiota. Nutr Res Rev 2013;26:191-209.
17. Zhou C, Gao L, Flaws JA. Exposure to an environmentally relevant phthalate mixture causes transgenerational effects on female reproduction in mice. Endocrinology 2017;158:1739-54.
18. Gill S, Brehm E, Leon K, Chiu J, Meling DD, Flaws JA. Prenatal exposure to an environmentally relevant phthalate mixture alters ovarian steroidogenesis and folliculogenesis in the F1 generation of adult female mice. Reprod Toxicol 2021;106:25-31.
19. Lee Y, Rattan S, Barakat R, et al. Early postnatal exposure to di(2-ethylhexyl) phthalate causes sex-specific disruption of gonadal development in pigs. Reprod Toxicol 2021;105:53-61.
20. National Research Council (US) Committee for the Update of the Guide for the Care and Use of Laboratory Animals. Guide for the care and use of laboratory animals, 8th edition. Washington (DC): National Academies Press (US); 2011. Available from: https://www.ncbi.nlm.nih.gov/books/NBK54050/. [Last accessed on 14 Aug 2024].
21. Den Braver-Sewradj SP, Piersma A, Hessel EVS. An update on the hazard of and exposure to diethyl hexyl phthalate (DEHP) alternatives used in medical devices. Crit Rev Toxicol 2020;50:650-72.
23. Chiang C, Lewis LR, Borkowski G, Flaws JA. Exposure to di(2-ethylhexyl) phthalate and diisononyl phthalate during adulthood disrupts hormones and ovarian folliculogenesis throughout the prime reproductive life of the mouse. Toxicol Appl Pharmacol 2020;393:114952.
24. Hartke JL, Monaco MH, Wheeler MB, Donovan SM. Effect of a short-term fast on intestinal disaccharidase activity and villus morphology of piglets suckling insulin-like growth factor-I transgenic sows. J Anim Sci 2005;83:2404-13.
25. Dudley MA, Jahoor F, Burrin DG, Reeds PJ. Brush-border disaccharidase synthesis in infant pigs measured in vivo with [2H3]leucine. Am J Physiol 1994;267:G1128-34.
26. Warner GR, Li Z, Houde ML, et al. Ovarian metabolism of an environmentally relevant phthalate mixture. Toxicol Sci 2019;169:246-59.
27. Li M, Bauer LL, Chen X, et al. Microbial composition and in vitro fermentation patterns of human milk oligosaccharides and prebiotics differ between formula-fed and sow-reared piglets. J Nutr 2012;142:681-9.
28. Callahan BJ, Wong J, Heiner C, et al. High-throughput amplicon sequencing of the full-length 16S rRNA gene with single-nucleotide resolution. Nucleic Acids Res 2019;47:e103.
29. Callahan BJ, McMurdie PJ, Rosen MJ, Han AW, Johnson AJ, Holmes SP. DADA2: high-resolution sample inference from Illumina amplicon data. Nat Methods 2016;13:581-3.
30. Bolyen E, Rideout JR, Dillon MR, et al. Reproducible, interactive, scalable and extensible microbiome data science using QIIME 2. Nat Biotechnol 2019;37:852-7.
31. Smith BN, Hannas M, Orso C, et al. Dietary osteopontin-enriched algal protein as nutritional support in weaned pigs infected with F18-fimbriated enterotoxigenic Escherichia coli. J Anim Sci 2020;98:skaa314.
32. Bokulich NA, Kaehler BD, Rideout JR, et al. Optimizing taxonomic classification of marker-gene amplicon sequences with QIIME 2’s q2-feature-classifier plugin. Microbiome 2018;6:90.
33. Pedregosa F, Varoquau, G, Gramfort A, et al. Scikit-learn: machine learning in python. J Mach Learn Res 2011;12:2825-30. Available from: https://dl.acm.org/doi/pdf/10.5555/1953048.2078195. [Last accessed on 14 Aug 2024]
34. Quast C, Pruesse E, Yilmaz P, et al. The SILVA ribosomal RNA gene database project: improved data processing and web-based tools. Nucleic Acids Res 2013;41:D590-6.
35. Love MI, Huber W, Anders S. Moderated estimation of fold change and dispersion for RNA-seq data with DESeq2. Genome Biol 2014;15:550.
36. Hagerty SL, Hutchison KE, Lowry CA, Bryan AD. An empirically derived method for measuring human gut microbiome alpha diversity: demonstrated utility in predicting health-related outcomes among a human clinical sample. PLoS One 2020;15:e0229204.
37. Rattan S, Brehm E, Gao L, Flaws JA. Di(2-Ethylhexyl) phthalate exposure during prenatal development causes adverse transgenerational effects on female fertility in mice. Toxicol Sci 2018;163:420-9.
38. Chiu K, Bashir ST, Gao L, et al. Subacute exposure to an environmentally relevant dose of di-(2-ethylhexyl) phthalate during gestation alters the cecal microbiome, but not pregnancy outcomes in mice. Toxics 2021;9:215.
39. de Freitas T, Zapaterini JR, Moreira CM, et al. Prenatal exposure to a mixture of different phthalates increases the risk of mammary carcinogenesis in F1 female offspring. Food Chem Toxicol 2021;156:112519.
40. Ljungvall K, Veeramachaneni DN, Hou M, Hultén F, Magnusson U. Morphology and morphometry of the reproductive organs in prepubertal and postpubertal male pigs exposed to di(2-ethylhexyl) phthalate before puberty: precocious development of bulbourethral glands. Theriogenology 2008;70:984-91.
41. Spjuth L, Saravia F, Johannisson A, Lundeheim N, Rodríguez-Martínez H. Effects of exposure of pre-pubertal boars to di(2-ethylhexyl) phthalate on their frozen-thawed sperm viability post-puberty. Andrologia 2006;38:186-94.
42. Su PH, Chang YZ, Chang HP, et al. Exposure to di(2-ethylhexyl) phthalate in premature neonates in a neonatal intensive care unit in Taiwan. Pediatr Crit Care Med 2012;13:671-7.
43. Yang YN, Yang YSH, Lin IH, et al. Phthalate exposure alters gut microbiota composition and IgM vaccine response in human newborns. Food Chem Toxicol 2019;132:110700.
44. Zhang YJ, Guo JL, Xue JC, Bai CL, Guo Y. Phthalate metabolites: characterization, toxicities, global distribution, and exposure assessment. Environ Pollut 2021;291:118106.
45. Wang G, Chen Q, Tian P, et al. Gut microbiota dysbiosis might be responsible to different toxicity caused by di-(2-ethylhexyl) phthalate exposure in murine rodents. Environ Pollut 2020;261:114164.
46. Yu J, Wang W, Wang J, Wang C, Li C. Short-term toxicity of dibutyl phthalate to mice intestinal tissue. Toxicol Ind Health 2019;35:20-31.
47. Yang TN, Li XN, Li XW, Li JY, Huang YQ, Li JL. DEHP triggers a damage severity grade increase in the jejunum in quail (Coturnix japonica) by disturbing nuclear xenobiotic receptors and the Nrf2-mediated defense response. Environ Toxicol Pharmacol 2022;96:104012.
48. Ahmed K, Kharoubi O, Aoues AEK, Bouchekara M, Khaladi B, Taleb M. Effect of gestational and lactational exposure to DEHP, DINP, and DEP on intestinal morphology, disaccharidases, and alkaline phosphatase in rats during postnatal development. Am J Perinatol 2018;35:1251-9.
49. Beumer J, Clevers H. Cell fate specification and differentiation in the adult mammalian intestine. Nat Rev Mol Cell Biol 2021;22:39-53.
50. Shum TF, Wang L, Chiou J. Impact of plasticizer on the intestinal epithelial integrity and tissue-repairing ability within cells in the proximity of the human gut microbiome. Int J Environ Res Public Health 2023;20:2152.
51. Chiu KK, Bashir ST, Abdel-Hamid AM, et al. Isolation of DiNP-degrading microbes from the mouse colon and the influence DiNP exposure has on the microbiota, intestinal integrity, and immune status of the colon. Toxics 2022;10:75.
52. Davis EC, Dinsmoor AM, Wang M, Donovan SM. Microbiome composition in pediatric populations from birth to adolescence: impact of diet and prebiotic and probiotic interventions. Dig Dis Sci 2020;65:706-22.
53. Wang M, Monaco MH, Donovan SM. Impact of early gut microbiota on immune and metabolic development and function. Semin Fetal Neonatal Med 2016;21:380-7.
54. Liu Z, Liu M, Wang H, et al. Glutamine attenuates bisphenol A-induced intestinal inflammation by regulating gut microbiota and TLR4-p38/MAPK-NF-κB pathway in piglets. Ecotoxicol Environ Saf 2024;270:115836.
55. Lei M, Menon R, Manteiga S, et al. Environmental chemical diethylhexyl phthalate alters intestinal microbiota community structure and metabolite profile in mice. mSystems 2019;4:e00724-19.
56. Cheng X, Chen J, Guo X, et al. Disrupting the gut microbiota/metabolites axis by di-(2-ethylhexyl) phthalate drives intestinal inflammation via AhR/NF-κB pathway in mice. Environ Pollut 2024;343:123232.
57. Wang M, Radlowski EC, Li M, Monaco MH, Donovan SM. Feeding mode, but not prebiotics, affects colonic microbiota composition and volatile fatty acid concentrations in sow-reared, formula-fed, and combination-fed piglets. J Nutr 2019;149:2156-63.
58. Fu X, Han H, Li Y, et al. Di-(2-ethylhexyl) phthalate exposure induces female reproductive toxicity and alters the intestinal microbiota community structure and fecal metabolite profile in mice. Environ Toxicol 2021;36:1226-42.
59. Quan J, Cai G, Ye J, et al. A global comparison of the microbiome compositions of three gut locations in commercial pigs with extreme feed conversion ratios. Sci Rep 2018;8:4536.
60. Waters JL, Ley RE. The human gut bacteria Christensenellaceae are widespread, heritable, and associated with health. BMC Biol 2019;17:83.
61. Goodrich JK, Waters JL, Poole AC, et al. Human genetics shape the gut microbiome. Cell 2014;159:789-99.
62. Lee-Sarwar KA, Kelly RS, Lasky-Su J, et al. Integrative analysis of the intestinal metabolome of childhood asthma. J Allergy Clin Immunol 2019;144:442-54.
63. Wang HG, Zhang MN, Wen X, et al. Cepharanthine ameliorates dextran sulphate sodium-induced colitis through modulating gut microbiota. Microb Biotechnol 2022;15:2208-22.
64. Chai X, Wen L, Song Y, et al. DEHP exposure elevated cardiovascular risk in obese mice by disturbing the arachidonic acid metabolism of gut microbiota. Sci Total Environ 2023;875:162615.
65. Wu X, Zhang T, Zhang T, Park S. The impact of gut microbiome enterotypes on ulcerative colitis: identifying key bacterial species and revealing species co-occurrence networks using machine learning. Gut Microbes 2024;16:2292254.
66. Tian Y, Fu M, Su J, et al. Gut microbiota dysbiosis and intestinal barrier impairment in diarrhea caused by cold drink and high-fat diet. Toxicology 2024;502:153728.
67. Nazina TN, Bidzhieva SK, Grouzdev DS, Löffler FE. Sphaerochaeta. In: Whitman WB, editor. Bergey’s manual of systematics of archaea and bacteria. Wiley; 2015. pp. 1-13.
68. Blaak EE, Canfora EE, Theis S, et al. Short chain fatty acids in human gut and metabolic health. Benef Microbes 2020;11:411-55.
69. Ghosh S, Pramanik S. Structural diversity, functional aspects and future therapeutic applications of human gut microbiome. Arch Microbiol 2021;203:5281-308.
70. El-Salhy M. Intestinal bacteria associated with irritable bowel syndrome and chronic fatigue. Neurogastroenterol Motil 2023;35:e14621.
71. Toubon G, Butel MJ, Rozé JC, et al. Association between gut microbiota at 3.5 years of age and body mass index at 5 years: results from two French nationwide birth cohorts. Int J Obes 2024;48:503-11.
Cite This Article
How to Cite
Monaco, M. H.; Coyne S.; Wang M.; Flaws J. A.; Irudayaraj J. M.; Cann I.; Donovan S. M. Effects of early life exposure to di(2-ethylhexyl) phthalate on jejunal morphology, sucrase activity, and colonic microbiota composition in young pigs. J. Environ. Expo. Assess. 2024, 3, 18. http://dx.doi.org/10.20517/jeea.2024.10
Download Citation
Export Citation File:
Type of Import
Tips on Downloading Citation
Citation Manager File Format
Type of Import
Direct Import: When the Direct Import option is selected (the default state), a dialogue box will give you the option to Save or Open the downloaded citation data. Choosing Open will either launch your citation manager or give you a choice of applications with which to use the metadata. The Save option saves the file locally for later use.
Indirect Import: When the Indirect Import option is selected, the metadata is displayed and may be copied and pasted as needed.
About This Article
Special Issue
Copyright
Data & Comments
Data
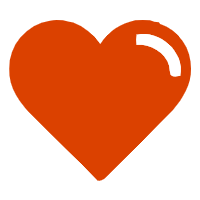
Comments
Comments must be written in English. Spam, offensive content, impersonation, and private information will not be permitted. If any comment is reported and identified as inappropriate content by OAE staff, the comment will be removed without notice. If you have any queries or need any help, please contact us at support@oaepublish.com.