SrSnO3 perovskite vs. Nd2Sn2O7 pyrochlores for oxidative coupling of methane: deciphering the reactive sites difference
Abstract
Herein, SrSnO3 perovskite and Nd2Sn2O7 pyrochlore with definite structures have been synthesized using a hydrothermal method, and the differences in their reactive sites for the oxidative coupling of methane (OCM) are investigated. The primary products of perovskite and pyrochlore are C2 hydrocarbons and COx, respectively. The C2 selectivity of perovskite is primarily affected by basic sites and chemisorbed oxygen species O22-. For pyrochlore, the key factors affecting methane conversion and COx selectivity are the acidic sites and reactive oxygen species (chemisorbed oxygen species and weakly bonded lattice oxygen). The chemisorbed oxygen species of pyrochlore are directly generated through intrinsic oxygen vacancies, whereas those of perovskite are generated through oxygen vacancies created under high-temperature lattice distortions. The tightness of the stacking between [SnO6] octahedra is the main factor affecting the acidic sites and oxygen vacancies of the two composite oxides. The stacking of [SnO6] octahedra in pyrochlore is loose, resulting in a relatively weak Sn–O bond strength. During the OCM reaction, the Sn–O bond is prone to breakage, resulting in abundant acidic sites and oxygen vacancies. Additionally, the influence of basic sites on the amount of chemisorbed oxygen species is more important than that of oxygen vacancies, which is attributed to the fact that basic sites can stabilize chemisorbed oxygen species on the catalyst surface.
Keywords
INTRODUCTION
Ethylene is widely used as a chemical intermediate to produce plastics and alpha-olefins, and methane is an abundant and relatively cheap natural resource[1,2]. The oxidative coupling of methane (OCM), a single-step reaction that converts methane to ethylene and ethane, has attracted significant attention in the past four decades[2,3]. Following the pioneering work of Keller and Bhasin, nearly thousands of types of catalysts have been developed for OCM[4], including alkali metal oxide modified alkaline earth metal oxides[5], rare earth metal oxides[6], Mn/Na2WO4/SiO2[7,8], and composite oxides such as perovskites and pyrochlores[9,10]. In particular, ABO3 perovskites and A2B2O7 pyrochlores are well known for their stable structures[11]. Their A- and B- site cations are easily replaceable, and thus, their surface acidity and basicity can be regulated. Furthermore, both these catalysts have excellent thermal stability, chemical stability, and oxygen ion mobility. Their phase structure is shown in Scheme 1. Considering that their active sites can meet most requirements for OCM, they are ideal catalysts for understanding the effect of active sites on this reaction[12,13]. ABO3 perovskites and A2B2O7 pyrochlores have the following similarities:
(1) A- and B- site cations have a stoichiometric ratio of 1:1.
(2) The A elements generally represent alkaline earth metal and rare earth metal cations, whereas B denotes transition metal cations that coordinate with six oxygen ions to form [BO6] octahedra[14-16].
(3) Their fine structures are regulated either by the tolerance factor (t)[17] or ionic radius ratio (rA/rB).
(4) The A–O bond is more ionic, and the B–O bond is more covalent[18,19].
ABO3 perovskites and A2B2O7 pyrochlores exhibit the following differences:
(1) A-site cations of perovskite are 12-coordinated, whereas those of pyrochlore are 8-coordinated[20,21].
(2) An ideal ABO3 perovskite exhibits a cubic structure, and it transforms into triclinic, monoclinic, rhombohedral, orthorhombic, and tetragonal crystal structures primarily due to cation displacement, octahedral distortions, and octahedral tilting that maintain corner-sharing connectivity. These structural deviations cause non-cubic perovskites to undergo phase transitions at high temperatures because they all tend to transition to cubic structures[17,22].
(3) The A2B2O7 composite oxide contains four sub-crystalline structures, namely layered perovskite (rA/rB > 1.78), pyrochlore (1.46 < rA/rB < 1.78), disorder defect fluorite (rA/rB ≈ 1.46), and rare earth C-type (rA/rB ≈ 1.17)[23,24]. The lattice disorder degree changes from ordered to disordered and then to ordered. Except for the layered perovskite phase structure, the other three sub-crystalline phases are cubic with intrinsic oxygen vacancies.
By comparing the similarities and differences between ABO3 and A2B2O7, we can understand the influence of their surface physicochemical properties on OCM and provide a theoretical basis for designing high-performance OCM catalysts. It has been found that Sn-based alkaline earth metal perovskites exhibit good OCM reaction performance, while Sn-based pyrochlore is commonly used in methane combustion reactions[25,26]. Petit et al. have prepared tin-based perovskite ASnO3 (A = Ca, Sr, Ba) for OCM reaction using SnO or SnCl4 as a precursor by sol-gel methods. It has shown that the C2 hydrocarbon selectivity of perovskites prepared by chlorine containing precursor SnCl4 is significantly higher than that of perovskites prepared by SnO as a precursor. The difference could be attributed to the increase in basicity of the catalyst surface due to the presence of bulk or surface Cl- species[25]. Yang et al. found that the OCM reaction performance of layered perovskites Sr2TiO4 and SrSn2O4 at 1,073 K was higher than that of corresponding SrTiO3 and SrSnO3 perovskites. The increase in methane conversion and C2 selectivity of layered perovskites is due to the O22- species produced by their decomposition under reaction conditions[27]. Park et al. synthesized Ln2Sn2O7 (Ln = La, Sm, and Gd) pyrochlores using a hydrothermal method for methane combustion reactions. It demonstrated that La2Sn2O7 has better catalytic activity than other catalysts. This is due to the lowest Sn–O bond energy in La2Sn2O7, which promotes the generation of more surface active sites[26]. Cheng et al. synthesized a series of La2CoxSn2-xO7-δ composite oxides with pyrochlore structure by co-precipitation method for methane combustion. They confirmed that with the increase of Co doping content, the strength of the Sn–O bond weakened, which promoted the formation of oxygen vacancies and, thus, benefited the activity of methane combustion[28].
In view of the above research, we are very curious about why tin-based perovskites exhibit OCM reaction activity, while tin-based pyrochlore mainly exhibits methane combustion activity. Comparing the similarities and differences in the crystal structure of the two composite oxides, this study addresses the following questions. How are the acid-base and redox properties of these two complex oxides different, and how do the presence and absence of intrinsic oxygen vacancies affect their oxygen properties? Accordingly, we synthesized two types of composite oxides, namely ASnO3 (A = Ca, Sr, Ba) and Ln2Sn2O7 (Ln = La, Pr, Nd), and the two catalysts with the best reaction performance (i.e., SrSnO3 and Nd2Sn2O7) were selected for detailed investigation of the variations in their reactive sites. By adopting various characterization techniques, the reasons for the differences in catalytic performance have been revealed, including the distinctions in major products and active sites.
EXPERIMENTAL
Catalyst preparation
Sr(NO3)2 (99.9%, Aladdin), Nd(NO3)3·6H2O (99.9%, Aladdin), SnCl4·5H2O (98.0%, Sinopharm Chemical Reagent Corporation, China), and KOH (99%, Sinopharm Chemical Reagent Corporation, China) were used for sample synthesis. That is, 24.0 mmol of A-site cation nitrate and 19.6 mmol of SnCl4·5H2O were added to 50 mL of deionized water. After stirring the mixed solution at room temperature for 1 h, 8 mol/L KOH was added to regulate the pH to 14. The resulting solution was stirred for 30 min, after which it was transferred to a 100 mL Teflon-lined stainless-steel autoclave and subjected to a hydrothermal reaction at 200 °C for 24 h. The resultant precipitate was washed with deionized water until the concentration of total dissolved solids (TDS) in the filtrate remained below 10 ppm. Subsequently, the precipitate was dried at
Catalyst characterization and reaction performance evaluation
The characterization and reaction performance testing conditions of the catalysts can be found in the Supplementary Materials.
RESULTS AND DISCUSSION
Catalytic performance
The catalytic performance of the ASnO3 (A = Ca, Sr, Ba) perovskite catalysts for OCM is shown in Supplementary Figure 1, demonstrating the following order based on CH4 conversion, C2 selectivity, and C2 yield at 600-650 °C: BaSnO3 >CaSnO3 > SrSnO3. However, after BaSnO3 achieves its maximum C2 yield at 700 °C, the catalytic performance shows the following order: SrSnO3 >CaSnO3 >BaSnO3. The O2 conversion is ~95% using BaSnO3 at 600 °C, whereas 100% O2 conversion is achieved using CaSnO3 or SrSnO3 at 800 °C. Below 800 °C, O2 conversion always obeys the following order: CaSnO3 > SrSnO3.
The catalytic performance of the Ln2Sn2O7 (Ln = La, Pr, Nd) catalysts for OCM is shown in Supplementary Figure 2. Supplementary Figure 2A shows that the CH4 conversion only changes slightly with increasing temperature, exhibiting the following order: Nd2Sn2O7 > Pr2Sn2O7 > La2Sn2O7. Supplementary Figure 2B shows that the O2 conversion is ~100% at 600 °C. With a rise in reaction temperature, oxygen is consumed completely; thus, the CH4 conversion changes slightly with an increase in temperature. As pyrochlores have an intrinsic oxygen vacancy[29,30], they achieve an oxygen conversion of ~100% at 600 °C. Supplementary Figure 2C shows that the COx selectivity of pyrochlores does not differ significantly with temperature changes. Based on their high reaction performance, we selected the SrSnO3 perovskite and Nd2Sn2O7 pyrochlore as the subjects for further study to determine their structure-reactivity relationships.
The results of SrSnO3 perovskite and Nd2Sn2O7 pyrochlore reaction performance with increasing temperature are shown in Figure 1A-D. With the rise in reaction temperature, the conversions of CH4 and O2, C2 selectivity, and C2 yield of SrSnO3 also escalate, and C2 hydrocarbon is the main product. SrSnO3 shows the best catalytic performance, achieving a CH4 conversion of 25.3%, O2 conversion of 99.9%, C2 selectivity of 55.7%, and C2 yield of 14.1%. Although the conversions of CH4 and O2 and the COx selectivity are less affected by temperature and the main product is COx, the C2 yield of the Nd2Sn2O7 catalyst is lower than 1% at 800 °C. The SrSnO3 perovskite with the best reaction performance underwent a long-term stability test at 800 oC for 100 h [Figure 1E]; the results showed that the reaction performance did not decrease.
Figure 1. OCM reaction performance of the SrSnO3 and Nd2Sn2O7 catalysts. (A) CH4 conversion; (B) O2 conversion; (C) C2 selectivity; and (D) C2 yield. (E) Long-term stability test of SrSnO3 catalyst at 800 °C for 100 h. Reaction conditions: CH4/O2/Ar = 4/1/5, WHSV = 18,000 mL·h-1·g-1. OCM: Oxidative coupling of methane; WHSV: weight hourly space velocity.
Temperature-programmed surface reaction (TPSR)-mass spectrometry (MS) was used in combination with online gas chromatography to investigate the effect of temperature on the catalytic performance of the two types of composite oxides for OCM reaction. Figure 2A shows that the main products (C2 hydrocarbons) are generated after OCM is performed using a SrSnO3 catalyst. Figure 2B shows that the primary reaction products are COx for Nd2Sn2O7 catalyst, and the amount of C2 hydrocarbons is extremely low. Table 1 shows that the onset temperature associated with deep methane oxidation is lower than the temperature required for the formation of C2 hydrocarbons, indicating that the two reactions may take place at different active sites.
Figure 2. CH4-TPSR-MS results in the presence of gaseous O2 over the (A) SrSnO3 and (B) Nd2Sn2O7. Reaction conditions:
Onset temperature of major products generated using different catalysts
Samples | Onset temperature of major products (oC) | |||
CO2 | CO | C2H4 | C2H6 | |
SrSnO3 | 575 | 470 | 660 | 660 |
Nd2Sn2O7 | 375 | 450 | 646 | 646 |
Structural identification
The description of the X-ray diffraction (XRD) analysis can be found in the Supplementary Materials. Supplementary Figures 3-5 and Supplementary Table 1 reveal that two different types of pure-phase composite oxide catalysts were synthesized, and there was no phase change due to the OCM reaction. All ASnO3 (A = Ca, Sr, Ba) and Ln2Sn2O7 (Ln = La, Pr, Nd) catalysts had a specific surface area of 5-12 m2/g, and there was no significant change after the reaction. The A/B atomic ratio was less than 1.00. The physicochemical properties of SrSnO3 and Nd2Sn2O7 composite oxide catalysts are shown in Table 2. SrSnO3 exhibits an orthorhombic crystalline phase, and Nd2Sn2O7 possesses a cubic crystalline phase.
Physico-chemical properties of the composite oxide catalysts
Samples | Crystalline phase | Lattice parameters | Specific surface areas (m2/g) | A/B atomic ratio | ||||
a (Å) | b (Å) | c (Å) | α, β, γ (o) | Fresh | Spent | |||
SrSnO3 | Orthorhombic | 5.710 | 5.723 | 8.067 | 90, 90, 90 | 6.4 | 5.9 | 0.94 |
Nd2Sn2O7 | Cubic | 10.576 | 10.576 | 10.576 | 90, 90, 90 | 10.5 | 9.9 | 0.95 |
Compared with XRD, Raman spectroscopy is a more sensitive probe of structural distortions, short-range order, and symmetry in solids, providing structural information concerning the long-range order of metal oxides[31]. Raman spectra of the fresh catalysts displayed in Supplementary Figure 6 and the attribution of their Raman modes shown in Supplementary Tables 2 and 3 further testify that these two series of pure phase composite oxides have been successfully synthesized. To investigate the crystalline phase transition of these catalysts under OCM reaction conditions, in situ Raman spectra have been obtained, and the results are displayed in Supplementary Figures 7 and 8, and Figure 3. The catalyst was pretreated at 800 °C under a pure He atmosphere for 30 min, which was then cooled to room temperature. Next, the CH4/O2/Ar = 4/1/5 gas was introduced, and Raman spectra of the catalysts were recorded at 25, 600, 700, and 800 °C, respectively.
The frequencies of Raman signals corresponding to SrSnO3 and Nd2Sn2O7 at room temperature are shown in Table 3[32,33]. Figure 3A shows that as the temperature increases, the Raman bands of SrSnO3 from 100 to 300 cm-1 gradually widen and disappear. Meanwhile, only one broadband is observed when the temperature exceeds 600 °C. Considering that the crystalline phase of SrSnO3 undergoes a transition from the Pnma orthorhombic phase to the Imma orthorhombic phase within this temperature range, the wide peak at 700-800 °C represents the Raman band of the Imma orthorhombic phase[34]. The change in symmetry of perovskite crystals may lead to lattice distortion, resulting in the generation of oxygen vacancies. Figure 3B shows that the Raman peaks of Nd2Sn2O7 pyrochlore mostly remain unchanged, except for the peak broadening caused by thermal lattice expansion at high temperatures. This indicates that the Nd2Sn2O7 pyrochlore does not undergo a phase transition or lattice distortion at temperatures required for OCM. Supplementary Figure 7 demonstrates that CaSnO3 did not undergo any crystal phase transition in the OCM reaction temperature range, while BaSnO3 underwent a crystal phase transition from defective BaSnO3 to regular cubic BaSnO3 accompanied by the disappearance of oxygen vacancies at temperatures above 700 oC. This well explains the decrease in reaction performance of BaSnO3 at temperatures above
Raman shifts (cm-1) of the active modes for SrSnO3 and Nd2Sn2O7 at room temperature
We have also provided the XRD pattern of SrSnO3 after stability testing in Supplementary Figure 10. The XRD pattern did not observe any diffraction peaks of other impurity peaks, indicating that the bulk structure of the perovskite is stable during stability testing.
Temperature-programmed desorption studies
As previously reported, the acid-base and oxygen properties on a catalyst surface are closely related to its catalytic performance for OCM[35-38]. Generally, moderate and strong acidic sites promote deep hydrocarbon oxidation, whereas moderate and strong basic sites favor C2 selectivity[39-41]. For different types of metal oxides, chemisorbed oxygen, such as O2-, O22-, O- and surface lattice O2-, may be considered the OCM-selective oxygen species[42-46]. To investigate the effect of these active sites on the catalytic performance for OCM, ASnO3 and Ln2Sn2O7 catalysts have been characterized using carbon dioxide temperature-programmed desorption (CO2-TPD), ammonia temperature-programmed desorption-mass spectrometry (NH3-TPD-MS), and oxygen temperature-programmed desorption-mass spectrometry (O2-TPD-MS). As shown in Supplementary Figures 11 and 12, and Supplementary Tables 4-6, as well as the discussion of the acid-base sites and reactive oxygen species of ASnO3 and Ln2Sn2O7 series in the supplementary materials, it can be concluded that it is reasonable to select SrSnO3 and Nd2Sn2O7 as typical examples of two composite oxides to explore surface reactive sites.
CO2-TPD profiles of SrSnO3 and Nd2Sn2O7 catalysts are shown in Figure 4A, and the quantitative results are listed in Supplementary Table 4. According to the number of moderate and strong basic sites (the CO2 desorption peak at 300-600 °C), SrSnO3 (0.10 μmol/m2) > Nd2Sn2O7 (0.01 μmol/m2), demonstrating that the number of basic sites favorable for the formation of C2 hydrocarbons is higher in perovskites than that in pyrochlores.
Figure 4. (A) CO2-TPD profiles; (B) NH3-TPD-MS profiles; and (C) O2-TPD-MS profiles. TPD: Temperature-programmed desorption; MS: mass spectrometry.
NH3-TPD-MS profiles of SrSnO3 and Nd2Sn2O7 are shown in Figure 4B, and the quantitative results are listed in Supplementary Table 5. Nd2Sn2O7 exhibits a higher number of moderate acidic sites (the NH3 desorption peak at 200-500 °C, 0.04 mmol/m2) than SrSnO3 (0.01 mmol/m2). Table 2 shows that the A/B atomic ratios of SrSnO3 and Nd2Sn2O7 are similar, indicating that the factors affecting their acidic and basic sites are unrelated to the enrichment of surface Sn elements.
O2-TPD-MS profiles of SrSnO3 and Nd2Sn2O7 are shown in Figure 4C, and the quantitative results are listed in Supplementary Table 6. Notably, SrSnO3 perovskite exhibits a higher desorption temperature and chemisorbs more oxygen species than Nd2Sn2O7 pyrochlores, indicating that both SrSnO3 and Nd2Sn2O7 have different mechanisms for activating gas-phase oxygen.
The results indicate that SrSnO3 with the perovskite phase has more abundant moderate and strong basic sites, chemisorbed oxygen species, and fewer moderate acidic sites than Nd2Sn2O7 with the pyrochlore phase.
Reactive oxygen species identification
To clarify the role of chemisorbed oxygen species in the OCM reaction, CH4-TPSR in the absence of O2 and continuous CH4-pulse tests have been conducted for SrSnO3 and Nd2Sn2O7 after the saturation of oxygen adsorption. In the TPSR tests, the two catalysts are treated with high-purity He to remove the impurities adsorbed on the catalyst surface, after which they are exposed to a 40% CH4/He mixture to perform a temperature-programmed reaction. Figure 5A and B shows that in the absence of gaseous O2, the main reaction product of SrSnO3 and Nd2Sn2O7 catalysts is CO2, indicating that the surface lattice oxygen species lead to deep methane oxidation. The amount of CO2 generated using SrSnO3 is significantly less than that produced using Nd2Sn2O7, indicating that the latter has a higher lattice oxygen content for deep methane oxidation than the former.
Figure 5. CH4-TPSR-MS results in the absence of gaseous O2 over (A) SrSnO3 and (B) Nd2Sn2O7. Reaction conditions: CH4/He = 4/6, WHSV = 18,000 mL·h-1·g-1. CH4-pulse tests performed over (C) SrSnO3 and (D) Nd2Sn2O7 after the saturation of oxygen adsorption. TPSR: Temperature-programmed surface reaction; MS: mass spectrometry; WHSV: .
In the continuous CH4-pulse tests, the two catalysts are pretreated using the same method. After adsorption saturation at 750 °C in a 10% O2/Ar gas flow (30 mL/min), 10% CH4-Ar is pulsed every 3 min through a
Furthermore, the types of reactive oxygen species related to SrSnO3 and Nd2Sn2O7 have been examined by in situ X-ray photoelectron spectroscopy (XPS). The XPS O1s spectra of the two composite oxides are measured at room temperature, and then the samples are exposed to a 10% O2-He gas mixture at 800 °C for 30 min. After being purged with high-purity He, the samples are allowed to cool to room temperature, and the spectra are collected again. As shown in Figure 6A and B, compared with the XPS O1s spectra obtained at room temperature, the peaks located at ~531 eV for both composite oxides decrease after the treatment with 10% O2-He at 800 °C, which can be attributed to the decomposition of carbonate or dehydration hydroxyl groups on the catalyst surface. The remaining peak located at ~531 eV can be ascribed to the O22- species[47]. In addition, the chemisorbed oxygen species on the surface of SrSnO3 are more abundant than those on Nd2Sn2O7.
Figure 6. In situ XPS O 1s spectra of the (A) SrSnO3 and (B) Nd2Sn2O7. XPS: X-ray photoelectron spectroscopy.
To investigate the reactive oxygen exchange mechanisms of the two catalysts, isotope 18O2 exchange experiments have been performed. As shown in Figure 7A and B, the products of both composite oxide catalysts are similar, indicating that the oxygen activation mechanisms of the two composite oxide catalysts are similar. Research has shown that the isotopic 18O2 exchange mechanism of metal oxides involves the following two pathways[48,49]:
Simple hetero-exchange mechanism:
Multiple hetero-exchange mechanism:
In the present study, the main products of both composite oxides are 16O16O, demonstrating that the activation of gas-phase oxygen in both composite oxides occurs via a multiple hetero-exchange mechanism. In addition, the generation of gas-phase oxygen 16O16O suggests the presence of binuclear oxygen species, such as O2- and O22[49], on the catalyst surface, which is consistent with the in situ XPS results, confirming that O22- is the chemisorbed oxygen species of these two composite oxides.
Overall, the results reveal that the chemisorbed selective oxygen species produced most by the two composite oxides in the OCM reaction process is O22-, which is activated through a multiple hetero-exchange mechanism.
Sn–O bond properties investigation
We use infrared (IR) spectra and XRD Rietveld refinement to investigate the Sn–O bond properties of both these composite oxides. In Figure 8A, the IR bands at 665 and 621 cm-1 are attributed to the stretching vibrations of the Sn–O bond for SrSnO3 and Nd2Sn2O7, respectively[33,50]. In addition, the IR peaks around 3,000 cm-1 are ascribed to surface hydroxyl groups[51], and the IR bands ranging from 1,000 to 1,500 cm-1 are related to surface carbonates[52,53]. Note that the IR peak intensity for the surface carbonate of SrSnO3 is significantly stronger than that of Nd2Sn2O7, which further suggests that the surface basic sites of SrSnO3 are more abundant than those of Nd2Sn2O7. Moreover, the B–O bond of A2B2O7 and ABO3 composite oxides have covalent bonding properties, making the B–O bond more prone to fracture than the A–O bond with ionic bonding properties[54,55]. From our calculations, the Sn–O bond force constant of Nd2Sn2O7 pyrochlore (3.162 N/cm) is lower than that of SrSnO3 perovskite (3.628 N/cm); the calculation method can be found in Supplementary Materials.
Figure 8. (A) FT-IR spectra; (B) H2-TPR profiles; and (C) EPR spectra of the catalysts. FT-IR: Fourier-transform infrared spectroscopy; TPR: ; EPR: electron paramagnetic resonance.
As shown in Supplementary Table 7, SrSnO3 has three different Sn–O bond lengths, which is due to their orthorhombic crystal phases and inclination, twisting, or distortion of BO6 octahedra, deviating from the cubic structure[56]. Conversely, the cubic Nd2Sn2O7 pyrochlore has one Sn–O bond length longer than that of perovskite, which is consistent with the fact that the Sn–O bond force constant of pyrochlore is smaller than that of perovskite. This conclusion can be further supported by the H2-temperature-programmed reduction (TPR) results. As shown in Figure 8B, no reduction peaks are observed for SrSnO3, but a peak attributed to the Sn–O bond reduction is observed around 500 °C for Nd2Sn2O7[57,58]. Supplementary Figure 13A confirms that after the H2-TPR reaction, the XRD diffraction peaks of SrSnO3 did not change, while the XRD pattern of Nd2Sn2O7 can be observed to belong to the diffraction peak of Nd2O3, indicating that the phase structure of SrSnO3 under a strong reducing atmosphere of H2 at high-temperature is stable, while Nd2Sn2O7 can be partially reduced. The XRD, Raman spectroscopy, and XPS results of the fresh catalysts indicate that no residual SnO2 content exists, and the H2-TPR results testify that the Sn–O lattice oxygen structure of Nd2Sn2O7 is looser than that of SrSnO3.
Previous studies have found that weaker B–O bonds in A2B2O7 composite oxides are easier to break, resulting in abundant oxygen vacancies[59]. Thus, Nd2Sn2O7 is expected to have more abundant oxygen vacancies than SrSnO3. In Figure 8C, the g value of 2.003 is attributed to the characteristic signal of oxygen vacancies[60,61], and the electron paramagnetic resonance (EPR) signal of Nd2Sn2O7 is stronger than that of SrSnO3, further demonstrating that Nd2Sn2O7 has more abundant oxygen vacancies than SrSnO3. EPR spectra of the spent catalysts displayed in Supplementary Figure 13B demonstrated that after OCM reaction, the oxygen vacancies of Nd2Sn2O7 are still more abundant than those in SrSnO3. Although Nd2Sn2O7 has more oxygen vacancies than SrSnO3, the previous results show that the amount of chemisorbed oxygen species in SrSnO3 is higher than that in Nd2Sn2O7. This indicates that the abundance of oxygen vacancies is not directly related to the amount of adsorbed oxygen species on the catalyst surface and may be influenced by other factors.
A brief discussion
During the OCM reaction, the SrSnO3 sample mainly contains C2 products, whereas the Nd2Sn2O7 sample primarily contains COx products. The analysis of acid-base properties reveals that the number of moderate and strong basic sites is greater in SrSnO3 than in Nd2Sn2O7. Meanwhile, the number of acidic sites is inversely proportional to that of basic sites. The O2-TPD-MS and in situ XPS O1s results indicate that their chemisorbed oxygen species (O22-) are OCM-selective oxygen species and the amount of chemisorbed oxygen and O22-/O2- ratios are greater for SrSnO3 than for Nd2Sn2O7, but the desorption temperature of SrSnO3 is higher than that of Nd2Sn2O7. In addition, the Sn–O bond strength of Nd2Sn2O7 is weaker than that of SrSnO3, resulting in more abundant oxygen vacancies in Nd2Sn2O7 than in SrSnO3, which contradicts the fact that the chemisorbed oxygen species on the surface of SrSnO3 are more abundant than Nd2Sn2O7.
Coordination unsaturated metal cations (acidic sites) are known to chemically adsorb the methyl radicals, ethyl radicals, and ethylene formed during OCM[62]. Consequently, a series of reactive oxygen species is generated via the activation of gas-phase oxygen, after which COx products are formed[62]. Therefore, the synergistic effect of acidic sites and reactive oxygen species (chemisorbed oxygen species or weakly bonded lattice oxygen) on the catalyst surface can lead to the generation of deep oxidation products. In addition, the weaker Sn–O bond in Nd2Sn2O7 is easier to break during the OCM reaction, resulting in the generation of more acidic sites. Basic sites are generally composed of hydroxyl groups and coordination unsaturated basic lattice oxygen species[53]. These electron-rich/basic sites can provide electrons to stabilize electron-deficient oxygen species such as O2-, O22-, and O-.
As illustrated in Supplementary Table 8, the electronegativity of Sr and Nd elements is lower than that of Sn element; compared with Sr–O, Nd–O, and Sn–O bonds, the tendency of electrons from Sr and Nd elements to transfer to O element is greater than that of Sn element. Therefore, Sr–O and Nd–O bonds exhibit ionic bond properties, while Sn–O bonds exhibit covalent bond properties. The electronegativity of Sr element is smaller than that of Nd and Sn elements; the electron transfer of Sr element in the Sr–O bond tends to be stronger than that of Nd element in the Nd–O and Sn element in the Sn–O bond towards O. Therefore, the lattice oxygen basicity of the Sr–O bond is stronger than that of the Nd–O and Sn–O bonds. In addition, the sum of the electronegativity of SrSnO3 metal elements (χSr + χSn = 2.91) is smaller than that of Nd2Sn2O7 (χSr + χSn = 3.10), the ability of SrSnO3 metal element electrons to transfer to oxygen is stronger than that of
Even though the surfaces of metal oxides are abundant in oxygen vacancies, which can create many electrophilic oxygen species, it is difficult to stabilize them on the surface when there are fewer basic sites[62]. This explains why, despite Nd2Sn2O7 having more oxygen vacancies than SrSnO3, there are fewer surface selective oxygen species O22- for Nd2Sn2O7 than for SrSnO3. Moreover, studies have shown that the temperature at which methane combustion occurs at acidic sites is lower than the OCM reaction at basic sites on metal oxide catalysts[41]. This explains why the onset temperature is lower for generating COx than for generating C2 products.
Considering the crystalline phase structure and high-temperature crystalline phase transition of the two composite oxides, it is important to note that Nd2Sn2O7 possesses intrinsic oxygen vacancies, and thus, the chemisorbed oxygen species are directly activated by oxygen vacancies, generating chemisorbed oxygen species at low temperatures. Therefore, the desorption temperature is lower for Nd2Sn2O7. At high temperatures, SrSnO3 undergoes lattice distortion[63], resulting in thermal defects such as oxygen vacancies. Hence, gas-phase oxygen is only activated to produce chemisorbed oxygen species at higher temperatures, resulting in a higher desorption temperature. The different desorption temperatures of chemisorbed oxygen species in the two composite oxides are attributed to their various gas-phase oxygen activation pathways.
The BO6 octahedra of perovskite are connected through corner-sharing to form a three-dimensional network [Scheme 2A][12], and the octahedra are stacked closely. According to the Pauling coordination polyhedron connection rules, the close corner-sharing of the octahedra leads to the maximum distance between positive cations and smaller Coulomb repulsion. In addition, this compact and symmetrical stacking mode makes the cations at the B-site subject to the Coulomb interaction between electrons, the effect of the surrounding oxygen ionic crystal field, and the spin-spin interaction within the atom, which balance each other to reach the most stable state. Compared with the close octahedra stacking of perovskite, the BO6 octahedra stacking of pyrochlore is looser and more disordered [Scheme 2B]. This disorderly and loose stacking mode leads to different effects on some B-site cations, and they cannot balance each other. Therefore, the B–O bond of pyrochlore is weaker than that of perovskite, and it is easier to break.
Scheme 2. The network of corner-sharing octahedra BO6 formed in (A) perovskite and (B) pyrochlore structure.
From the results, the variations in the degrees of lattice oxygen relaxation and acidic sites between perovskite and pyrochlore may be caused by the different packing modes of BO6 octahedra. BO6 octahedra in perovskite are more tightly packed than those in pyrochlore. Therefore, the B–O bond in perovskite is less easily reduced and less easily broken to produce acidic sites than that in pyrochlore.
CONCLUSIONS
In summary, we synthesized two types of composite oxides (SrSnO3 and Nd2Sn2O7) with definite structures via a hydrothermal method for OCM catalysis. Furthermore, we compared and analyzed the differences in their active sites using various characterization methods. The SrSnO3 perovskite catalyst mainly produces C2 hydrocarbons during the OCM reaction, whereas the Nd2Sn2O7 pyrochlore catalyst primarily generates COx products.
SrSnO3 perovskite has abundant basic sites that produce a synergistic effect with chemisorbed oxygen species of O22-, which, in turn, facilitates the formation of C2 products. The thermal defects generated by the high-temperature lattice distortion of perovskites (such as oxygen vacancies that lead to the formation of chemisorbed oxygen species) play an important role in the generation of C2 hydrocarbons. When an appropriate number of basic sites exist along with abundant chemisorbed oxygen species, perovskites exhibit a high selectivity and yield of C2. For Nd2Sn2O7 pyrochlore, the synergistic effect of the abundant acidic sites on their surfaces and the reactive oxygen species (chemisorbed oxygen species) generated by intrinsic oxygen vacancies and weakly bonded lattice oxygen mainly results in the formation of COx products. The Sn–O bond strength of pyrochlore is the main factor affecting the formation of deep oxidation products (COx). The weaker the Sn–O bond, the higher the number of acid sites, and the higher the COx selectivity.
The oxygen vacancies of both composite oxides are related to the Sn–O bonds. The weaker the Sn–O bond of the composite oxides, the richer the oxygen vacancies. However, the amount of chemisorbed oxygen species is not only related to oxygen vacancies but also to basic sites. Composite oxides generally have abundant oxygen vacancies and fewer basic sites, resulting in a lower amount of electrophilic oxygen species stabilized on the catalyst surface. Basic sites have a stronger impact on the amount of chemisorbed oxygen species than oxygen vacancies.
The Sn–O bond strength and the compactness of the [SnO6] octahedron are responsible for the differences in the catalytic performance of SrSnO3 and Nd2Sn2O7 for OCM. Pyrochlores have a weak Sn–O bond strength, and their [SnO6] octahedra are loosely stacked. Thus, Sn–O bonds break easily and acidic sites are readily generated. Moreover, because lattice oxygen can be easily reduced and its mobility is high, COx products are primarily formed in pyrochlores; meanwhile, contrasting results are observed for perovskites.
DECLARATIONS
Authors’ contributions
Data curation, investigation, software: Ouyang R, Zhong Xs, Gong Y, Liu Y
Project administration, supervision, software: Fang X, Shen J
Conceptualization, funding acquisition, investigation, methodology, project administration, resources, supervision, validation, writing - review and editing: Wang X, Xu J
Availability of data and materials
Detailed experimental procedures and related data were published as Supplementary Materials in the journal, and the data supporting the findings of this study are available within its Supplementary Materials.
Financial support and sponsorship
This work was supported by the National Natural Science Foundation of China (22172071, 22102069, 22376090, 22262021, 22362026) and the Natural Science Foundation of Jiangxi Province (20224BAB213017).
Conflicts of interest
All authors declared that there are no conflicts of interest.
Ethical approval and consent to participate
Not applicable.
Consent for publication
Not applicable.
Copyright
© The Author(s) 2024.
Supplementary Materials
REFERENCES
1. Doherty CJ, Kay SA. Circadian surprise - it’s not all about transcription. Science 2012;338:338-40.
3. Qiu X, Wong N, Tin K, Zhu Q. Review low temperature catalysts for oxidative coupling of methane. J Chem Technol Biotechnol 1996;65:303-8.
4. Thybaut J, Marin G, Mirodatos C, et al. A novel technology for natural gas conversion by means of integrated oxidative coupling and dry reforming of methane. Chem Ing Tech 2014;86:1855-70.
5. Aljama H, Nørskov JK, Abild-pedersen F. Tuning methane activation chemistry on alkaline earth metal oxides by doping. J Phys Chem C 2018;122:22544-8.
6. Zhou X, Pang Y, Liu Z, et al. Active oxygen center in oxidative coupling of methane on La2O3 catalyst. J Energy Chem 2021;60:649-59.
7. Si J, Sun W, Zhao G, Lu Y. Support oxide tuning of MnOx-Na2WO4 catalysts enables low-temperature light-off of OCM. Fuel 2022;311:122539.
8. Hiyoshi N, Ikeda T. Oxidative coupling of methane over alkali chloride–Mn–Na2WO4/SiO2 catalysts: promoting effect of molten alkali chloride. Fuel Process Technol 2015;133:29-34.
9. Lim S, Choi J, Jin Suh D, Lee U, Song KH, Ha J. Low-temperature oxidative coupling of methane using alkaline earth metal oxide-supported perovskites. Catal Today 2020;352:127-33.
10. Roger A, Petit C, Kiennemann A. Effect of metallo-organic precursors on the synthesis of Sm–Sn pyrochlore catalysts: application to the oxidative coupling of methane. J Catal 1997;167:447-59.
11. Murthy PR, Liu Y, Wu G, Diao Y, Shi C. Oxidative coupling of methane: perspective for high-value C2 chemicals. Crystals 2021;11:1011.
12. Mi J, Chen J, Chen X, Liu X, Li J. Recent status and developments of vacancies modulation in the ABO3 perovskites for catalytic applications. Chemistry 2023;29:e202202713.
13. Deng J, Chen P, Xia S, et al. Advances in oxidative coupling of methane. Atmosphere 2023;14:1538.
14. Fisher JG, Rout D, Moon K, Kang SL. High-temperature X-ray diffraction and Raman spectroscopy study of (K0.5Na0.5)NbO3 ceramics sintered in oxidizing and reducing atmospheres. Mater Chem Phys 2010;120:263-71.
15. Kapusta B, Guillopé M. Molecular dynamics study of the perovskite MgSiO3 at high temperature: structural, elastic and thermodynamical properties. Phys Earth Planet In 1993;75:205-24.
16. Gao L, Liang K, Liu Z, Chen H, Zhang J. Structure dependence of dielectric properties in Ca-doped bismuth magnesium niobate pyrochlores. J Alloys Compd 2022;922:165859.
17. Muñoz HJ, Korili SA, Gil A. Progress and recent strategies in the synthesis and catalytic applications of perovskites based on lanthanum and aluminum. Materials 2022;15:3288.
18. Renju UA, Prabhakar Rao P, Vaisakhan Thampi DS. Influence of phase transition from order to disorder and Philip’s ionicity on the thermal expansion coefficient of pyrochlore type compositions with a multivalent environment. New J Chem 2017;41:245-55.
19. Behara S, Poonawala T, Thomas T. Crystal structure classification in ABO3 perovskites via machine learning. Comput Mater Sci 2021;188:110191.
20. Liu H, Cheng J, Dong H, et al. Screening stable and metastable ABO3 perovskites using machine learning and the materials project. Comput Mater Sci 2020;177:109614.
21. Jerez A, López ML, García-Martín S, Veiga ML, Pico C. Defect pyrochlore structure A2B2X6: a general approach to the coordination polyhedra around the metal ions. J Mater Sci 1991;26:5163-6.
22. Jerpoth SS, Iannello J, Aboagye EA, Yenkie KM. Computer-aided synthesis of cost-effective perovskite crystals: an emerging alternative to silicon solar cells. Clean Technol Envir 2020;22:1187-98.
23. Subramanian M, Aravamudan G, Subba Rao G. Oxide pyrochlores - A review. Prog Solid State Ch 1983;15:55-143.
24. Shamblin J, Tracy CL, Ewing RC, et al. Structural response of titanate pyrochlores to swift heavy ion irradiation. Acta Mater 2016;117:207-15.
25. Petit C, Teymouri M, Roger A, Rehspringer J, Hilaire L, Kiennemann A. Preparation and characterization of ASnO3 (A=Ca, Sr or Ba) tin compounds for methane oxidative coupling. Stud Surf Sci Catal 1995;91:607-16.
26. Park S, Hwang HJ, Moon J. Catalytic combustion of methane over rare earth stannate pyrochlore. Catal Lett 2003;87:219-23.
27. Yang W, Yan Q, Fu X. Oxidative coupling of methane over Sr−Ti, Sr−Sn perovskites and corresponding layered perovskites. React Kinet Catal Lett 1995;54:21-7.
28. Cheng J, Wang H, Hao Z, Wang S. Catalytic combustion of methane over cobalt doped lanthanum stannate pyrochlore oxide. Catal Commun 2008;9:690-5.
29. Lumpkin GR, Aughterson RD. Perspectives on pyrochlores, defect fluorites, and related compounds: building blocks for chemical diversity and functionality. Front Chem 2021;9:778140.
30. Beyerlein R, Horowitz H, Longo J, Leonowicz M, Jorgensen J, Rotella F. Neutron diffraction investigation of ordered oxygen vacancies in the defect pyrochlores, Pb2Ru2O6.5 and PbT1Nb2O6.5. J Solid State Chem 1984;51:253-65.
31. Moncada J, Adams WR, Thakur R, Julin M, Carrero CA. Developing a Raman spectrokinetic approach to gain insights into the structure–reactivity relationship of supported metal oxide catalysts. ACS Catal 2018;8:8976-86.
32. Alammar T, Hamm I, Grasmik V, Wark M, Mudring AV. Microwave-assisted synthesis of perovskite SrSnO3 nanocrystals in ionic liquids for photocatalytic applications. Inorg Chem 2017;56:6920-32.
33. Rajakumaran R, Balamurugan K, Chen SM, Sukanya R. Facile synthesis of neodymium stannate nanoparticles an effective electrocatalyst for the selective detection of dimetridazole in biological samples. Anal Chim Acta 2022;1190:339234.
34. Cortés-adasme E, Castillo R, Conejeros S, Vega M, Llanos J. Behavior of Eu ions in SrSnO3: optical properties, XPS experiments and DFT calculations. J Alloys Compd 2019;771:162-8.
35. Wu T, Wei Y, Xiong J, et al. Atomically dispersed SrOx species on exposed {2 2 2} facets of pyrochlore La2Zr2O7 nanocrystals for boosting low-temperature oxidative coupling of methane. Fuel 2023;333:126479.
36. Kim I, Lee G, Na HB, Ha J, Jung JC. Selective oxygen species for the oxidative coupling of methane. Mol Catal 2017;435:13-23.
37. Thum L, Rudolph M, Schomäcker R, et al. Oxygen activation in oxidative coupling of methane on calcium oxide. J Phys Chem C 2019;123:8018-26.
38. Jiang S, Ding W, Zhao K, et al. Enhanced chemical looping oxidative coupling of methane by Na-doped LaMnO3 redox catalysts. Fuel 2021;299:120932.
39. Yan L, Zhang J, Gao X, et al. Oxidative coupling of methane over Mo-Sn catalysts. Chem Commun 2021;57:13297-300.
40. Zhao M, Ke S, Wu H, Xia W, Wan H. Flower-like Sr-La2O3 microspheres with hierarchically porous structures for oxidative coupling of methane. Ind Eng Chem Res 2019;58:22847-56.
41. Papa F, Luminita P, Osiceanu P, Birjega R, Akane M, Balint I. Acid–base properties of the active sites responsible for C2+ and CO2 formation over MO–Sm2O3 (M=Zn, Mg, Ca and Sr) mixed oxides in OCM reaction. J Mol Catal A Chem 2011;346:46-54.
42. Gambo Y, Jalil A, Triwahyono S, Abdulrasheed A. Recent advances and future prospect in catalysts for oxidative coupling of methane to ethylene: a review. J Ind Eng Chem 2018;59:218-29.
43. Gu S, Oh H, Choi J, et al. Effects of metal or metal oxide additives on oxidative coupling of methane using Na2WO4/SiO2 catalysts: reducibility of metal additives to manipulate the catalytic activity. Appl Catal A Gen 2018;562:114-9.
44. Yoon S, Lim S, Choi JW, Suh DJ, Song KH, Ha JM. Study on the unsteady state oxidative coupling of methane: effects of oxygen species from O2, surface lattice oxygen, and CO2 on the C2+ selectivity. RSC Adv 2020;10:35889-97.
45. Zanina A, Kondratenko VA, Lund H, et al. The role of adsorbed and lattice oxygen species in product formation in the oxidative coupling of methane over M2WO4/SiO2 (M = Na, K, Rb, Cs). ACS Catal 2022;12:15361-72.
46. Tang L, Yamaguchi D, Wong L, Burke N, Chiang K. The promoting effect of ceria on Li/MgO catalysts for the oxidative coupling of methane. Catal Today 2011;178:172-80.
47. Pang Y, Zhou X, Vovk EI, et al. Understanding lanthanum oxide surface structure by DFT simulation of oxygen 1s calibrated binding energy in XPS after in situ treatment. Appl Surf Sci 2021;548:149214.
48. Doornkamp C, Clement M, Ponec V. The isotopic exchange reaction of oxygen on metal oxides. J Catal 1999;182:390-9.
49. Martin D, Duprez D. Mobility of surface species on oxides. 1. Isotopic exchange of 18O2 with 16O of SiO2, Al2O3, ZrO2, MgO, CeO2, and CeO2-Al2O3. Activation by noble metals. Correlation with oxide basicity. J Phys Chem 1996;100:9429-38.
50. Chandra S, Ravichandran K, George G, Arun T, Rajkumar PV. Influence of Fe and Fe+F doping on the properties of sprayed SnO2 thin films. J Mater Sci Mater Electron 2016;27:9558-64.
51. Lopes LB, Vieira LH, Assaf JM, Assaf EM. Effect of Mg substitution on LaTi1-x MgxO3+δ catalysts for improving the C2 selectivity of the oxidative coupling of methane. Catal Sci Technol 2021;11:283-96.
52. Gao W, Zhou T, Wang Q. Controlled synthesis of MgO with diverse basic sites and its CO2 capture mechanism under different adsorption conditions. Chem Eng J 2018;336:710-20.
53. Davydov A, Shepotko M, Budneva A. Basic sites on the oxide surfaces: their effect on the catalytic methane coupling. Catal Today 1995;24:225-30.
54. Liu C, Li Y, Li Y, et al. First principle calculation of helium in La2Zr2O7: effects on structural, electronic properties and radiation tolerance. J Nucl Mater 2018;500:72-80.
55. Srivastava AM, Brik MG, Beers WW, Cohen WE. Luminescence of Mn4+ in the orthorhombic perovskites, AZrO3 (A=Ca, Sr). Opt Mater 2021;114:110906.
56. Antonio J, Muñoz H, Rosas-huerta J, et al. Effects of the phase transition on the structural, mechanical, electronic and vibrational properties of the CaSnO3 perovskite: study under hydrostatic pressure. J Phys Chem Solids 2022;163:110594.
57. Wang H, Huang H, Bashir K, Li C. Isolated Sn on mesoporous silica as a highly stable and selective catalyst for the propane dehydrogenation. Appl Catal A Gen 2020;590:117291.
58. Salmones J, Wang J, Galicia JA, Aguilar-rios G. H2 reduction behaviors and catalytic performance of bimetallic tin-modified platinum catalysts for propane dehydrogenation. J Mol Catal A Chem 2002;184:203-13.
59. Petit C, Rehspringer JL, Kaddouri A, Libs S, Poix P, Kiennemann A. Oxidative coupling of methane by pyrochlore oxide A2B2O7 (A = rare earth, B = Ti, Zr, Sn). Relation between C2 selectivity and B-O bond energy. Catal Today 1992;13:409-16.
60. Zhang C, Liu X, Jiang M, Wen Y, Zhang J, Qian G. A review on identification, quantification, and transformation of active species in SCR by EPR spectroscopy. Environ Sci Pollut Res Int 2023;30:28550-62.
61. Shi Y, Wang X, Chen L, et al. In situ DRIFT study on NH3 selective catalytic reduction of NOx over HBEA zeolite doped with CeO2. Appl Surf Sci 2020;506:144715.
62. Choudhary V. Acidity/basicity of rare-earth oxides and their catalytic activity in oxidative coupling of methane to C2-hydrocarbons. J Catal 1991;130:411-22.
Cite This Article
How to Cite
Ouyang, R.; Xu, J.; Zhong, X.; Gong, Y.; Liu, Y.; Fang, X.; Shen, J.; Wang, X. SrSnO3 perovskite vs. Nd2Sn2O7 pyrochlores for oxidative coupling of methane: deciphering the reactive sites difference. Chem. Synth. 2024, 4, 72. http://dx.doi.org/10.20517/cs.2024.29
Download Citation
Export Citation File:
Type of Import
Tips on Downloading Citation
Citation Manager File Format
Type of Import
Direct Import: When the Direct Import option is selected (the default state), a dialogue box will give you the option to Save or Open the downloaded citation data. Choosing Open will either launch your citation manager or give you a choice of applications with which to use the metadata. The Save option saves the file locally for later use.
Indirect Import: When the Indirect Import option is selected, the metadata is displayed and may be copied and pasted as needed.
About This Article
Special Issue
Copyright
Author Biographies
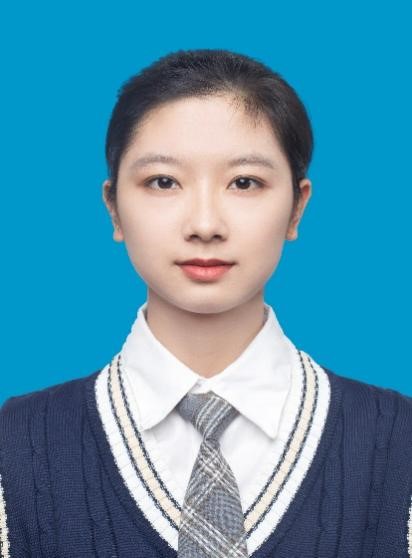
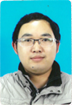
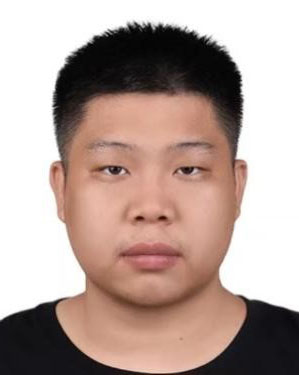
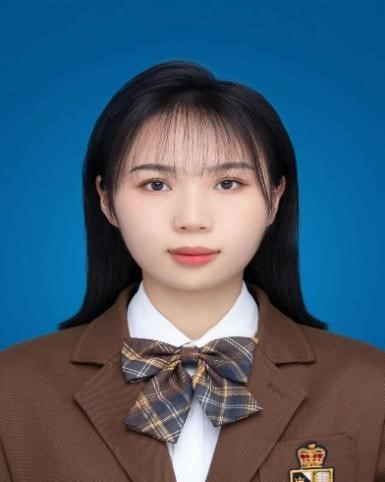
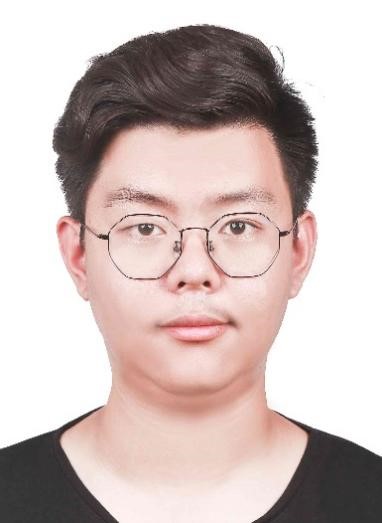
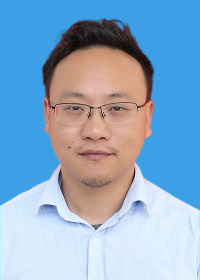
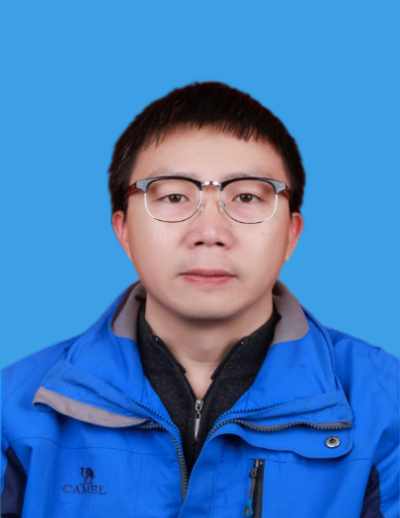
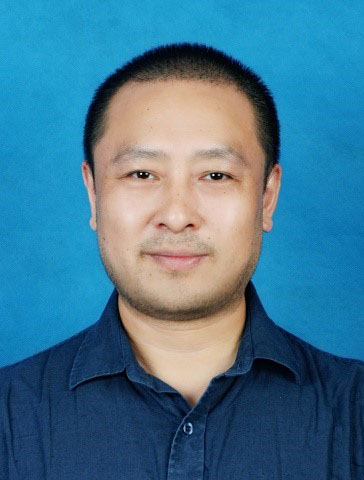
Comments
Comments must be written in English. Spam, offensive content, impersonation, and private information will not be permitted. If any comment is reported and identified as inappropriate content by OAE staff, the comment will be removed without notice. If you have any queries or need any help, please contact us at support@oaepublish.com.