Synthesis of metal-phenanthroline-modified hypercrosslinked polymer for enhanced CO2 capture and conversion via chemical and photocatalytic methods under ambient conditions
Abstract
Catalytic conversion of CO2 into valuable chemicals is a promising approach to mitigate the greenhouse effect and alleviate energy shortages. Hypercrosslinked polymers (HCPs) offer a scalable and stable platform for this conversion, but they often suffer from low CO2 adsorption and activation capabilities, necessitating high temperatures and pressures for effectiveness. To overcome these limitations, nitrogen-based CO2-philic active sites have been integrated into the structure of HCPs, enhancing CO2 attraction and leading to superior adsorption performance. The incorporation of cobalt ions further bolsters CO2 affinity, with HCP-PNTL-Co-B achieving the highest observed adsorption heat of 33.0 kJ·mol-1 alongside a substantial 2.0 mmol·g-1 CO2 uptake. These modified HCPs exhibit higher yields and reaction rates in cycloaddition reactions with cocatalyst tetrabutylammonium bromide at room temperature and atmospheric pressure, while HCP-1,10-phenanthroline (PNTL)-Co-B demonstrates a higher CO production rate (2,173 μmol·g-1·h-1) and selectivity (84%) in photocatalytic reduction reaction. This research has successfully achieved outstanding carbon dioxide capture and conversion performance at room temperature and atmospheric pressure by introducing CO2-philic active sites and cobalt ions into HCPs via facile one-step polymerization. This study provides a new method to design highly efficient organic catalysts for CO2 conversion.
Keywords
INTRODUCTION
The transformation of CO2 into value-added chemicals offers a dual benefit: it reduces greenhouse gas emissions and recycles carbon resources[1-6]. The domain of CO2 catalytic conversion has seen the emergence of numerous homogeneous and heterogeneous catalysts[7-11]. Heterogeneous catalysts, including carbon-based materials[12,13], silica[14], metal-organic frameworks (MOFs)[15-17], covalent organic frameworks (COFs)[18,19], and porous organic frameworks (POPs)[20-24], are preferred over their homogeneous counterparts due to their ease of separation, purification, and recyclability. Hypercrosslinked polymers (HCPs), as a subcategory of porous organic polymers, are particularly notable for their high surface area, diverse porosity, affordability, facile functionalization, and robust stability[25-31]. Moreover, HCPs are adept at incorporating catalytic sites, facilitating the creation of multiphase catalytic systems, which positions them as strong candidates for CO2 conversion applications[32-39].
At mild conditions, the effectiveness of CO2 conversion is closely tied to the material’s CO2 capture aptitude, with materials exhibiting low adsorption capacities facing limitations due to reduced CO2 concentrations at catalytic sites[40-42]. Unfortunately, most metal-based HCPs reported to date possess suboptimal CO2 adsorption capabilities, leading to inefficient utilization of CO2 under ambient conditions[30,32,37,43]. Beyond merely enhancing the CO2 adsorption capacity to improve conversion[32,44,45], scant research addresses the interaction between CO2 and the catalytic material. Integrating CO2-philic sites, such as nitrogen atoms or nitrogen-functional groups, can bolster the adsorption force for CO2, amplify adsorption capacity, and concentrate CO2 around catalytic sites[46-49]. This process not only increases the concentration of CO2 but also activates the inert CO2 molecules adsorbed on the surface, thereby improving the conversion efficiency. Nonetheless, the post-functionalization approach for introducing CO2-philic sites is often labor-intensive and may compromise the integrity of the porous structures of HCPs. It also suffers from the uneven distribution of CO2-philic sites[50-52]. Alternatively, synthesizing HCPs through hypercrosslinking polymerization with monomers already containing CO2-philic sites presents a solution, potentially enabling effective CO2 conversion in ambient conditions.
Motivated by the need to improve interactions with CO2 and stabilize metal ion catalytic sites, we utilized 1,10-phenanthroline (PNTL), a nitrogen-rich ligand known for its ability to enhance the binding with acidic CO2 molecules and coordinate with metal ions[53]. We synthesized PNTL-based HCPs through the Friedel-Crafts alkylation, employing derivatives of PNTL as functional monomers [Scheme 1]. This approach strategically increased the nitrogen content within the HCP framework, circumventing the issues of inhomogeneous CO2-philic site distribution often encountered with post-functionalization. Subsequently, we created a composite catalytic system, HCP-PNTL-Co, by introducing cobalt ions (Co2+) through coordination. This system was applied to the cycloaddition of CO2 with epoxides and the photoreduction of CO2 under ambient conditions. Among the different types, HCP-PNTL-Co-B, with the highest nitrogen content of 5.6 wt%, demonstrated superior CO2 adsorption, reaching 2.0 mmol/g at 273 K and 1 bar, and an adsorption heat of 33.0 kJ/mol. This material outperformed HCP-PNTL-Co-A, which had a lower nitrogen content of 2.9 wt%, showing increased catalytic activity and efficiency in both the cycloaddition and photoreduction reactions at ambient conditions. This study illustrates that the direct introduction of CO2-philic active sites into HCPs significantly augments both CO2 adsorption capacity and activation potential, avoiding the need for post-functionalization and ensuring a more uniform distribution of CO2-philic active sites, thus facilitating the catalytic conversion of CO2. These findings highlight the potential for efficient CO2 catalytic conversion, offering a promising approach to addressing the challenges of greenhouse gas emissions and energy scarcity and paving the way for developing and designing new strategies for efficient CO2 catalytic conversion systems.
EXPERIMENTAL
Materials
Acetonitrile (MeCN), [Gas Chromatography (GC)], 1,3,5-trimethylbenzene (GC), formaldehyde dimethyl acetal, (3,5-diphenylphenyl)boronic acid (98%), 3,8-dibromo-1,10-phenanthroline (98%), (4-(9H-carbazol-9-yl)phenyl)boronic acid (98%), tetrakis (triphenylphosphine) palladium (99%), cobalt chloride hexahydrate [Analytical Reagent (AR)], 4-dimethylaminopyridine (99%), tetraphenylphosphonium bromide (TPPB) (99%), epoxides (AR), triethanolamine (TEOA) (AR), and tris(2,2’-bipyridyl)ruthenium(II) chloride hexahydrate (98%) were purchased from Aladdin Chemical Reagent Corp. Tetrabutylammonium bromide (TBAB) (AR), dichloromethane (AR), 1,2-dichloroethane (AR), HCl (37%), 1,4-dioxane (AR), N,N-dimethylformamide (AR), ethylacetate (AR), tetrahydrofuran (AR), anhydrous potassium carbonate (AR), magnesium sulfate anhydrous (AR), iron(III) chloride anhydrous [Chemical Reagent (CR)], ethanol (AR), and methanol (AR) were provided from Sinopharm Chemical Reagent Co., Ltd.
Synthesis procedure
Synthesis of porous polymers
The HCP-PNTL-A[54] was synthesized by adding PNTL-A, formaldehyde dimethyl acetal, and 1,2-dichloroethane to a glass flask. Anhydrous FeCl3 was then introduced under a nitrogen atmosphere, followed by stirring at 45 °C for 5 h and 80 °C for 19 h. The resulting solid polymer was collected, washed with methanol and deionized (DI) water, and extracted with methanol using a Soxhlet apparatus. The dried polymer was obtained as a brown solid. The procedure for HCP-PNTL-B[55] was similar, replacing PNTL-A with PNTL-B.
Synthesis of metal-containing polymers
For HCP-PNTL-Co-A synthesis, a mixture of HCP-PNTL-A, cobalt chloride, and MeCN was stirred and refluxed at 65 °C for 12 h under nitrogen. After purification, the product was obtained as a solid. HCP-PNTL-Co-B was synthesized using the same method with HCP-PNTL-B instead of HCP-PNTL-A.
The yields of HCP-PNTL-A, HCP-PNTL-B, HCP-PNTL-Co-A, and HCP-PNTL-Co-B were 132%, 126%, 90%, and 85%, respectively.
RESULTS AND DISCUSSION
Structural and morphological characterizations
Confirmation of successful material synthesis was achieved through Fourier-transform infrared spectroscopy (FT-IR), solid-state 13C cross-polarization/magic angle spinning nuclear magnetic resonance (13C CP/MAS NMR), and elemental analysis (EA). The FT-IR spectra [Figure 1A and B] displayed characteristic absorption peaks at 1,597 and 1,250 cm-1, indicative of the stretching vibrations of C=N and
Figure 1. FT-IR spectra (A) and (B), solid-state 13C NMR spectra (C), TGA (D) (measured under N2 atmosphere), and solid-state UV-visible absorption spectra (E) and (F) of HCP-PNTL and HCP-PNTL-Co. FT-IR: Fourier-transform infrared spectroscopy; NMR: nuclear magnetic resonance; TGA: thermogravimetric analysis; UV: ultraviolet; HCP: hypercrosslinked polymer; PNTL: 1,10-phenanthroline.
The HCPs are demonstrated to possess irregular layered structures by scanning (SEM) and transmission electron microscopy (TEM) images [Supplementary Figure 1]. A uniform distribution of carbon (C), nitrogen (N), and cobalt (Co) within the HCP-PNTL-Co samples is indicated by elemental mapping
Porosity characteristics and gas adsorption capacities
The internal porosity of HCP-PNTL and HCP-PNTL-Co was assessed using nitrogen adsorption and desorption isotherms at 77.3 K. Figure 3A shows that the N2 uptake of HCP-PNTL-A and HCP-PNTL-B sharply increased at low relative pressure (P/P0 < 0.001), indicating their microporous nature. The significant desorption hysteresis at intermediate pressure suggests the presence of mesopores. Upon loading with cobalt metal ions, the nitrogen uptake of HCP-PNTL-Co was notably lower than that of HCP-PNTL [Figure 3A], indicating pore blockage. The Brunauer-Emmett-Teller (BET) surface areas were 951 m2·g-1 for HCP-PNTL-A and 475 m2·g-1 for HCP-PNTL-B, while they decreased to 609 m2·g-1 for HCP-PNTL-Co-A and 147 m2·g-1 for HCP-PNTL-Co-B [Supplementary Table 2]. Figure 3B depicts the pore size distribution calculated by nonlocal density functional theory (NLDFT), confirming the microporous and mesoporous structures of HCP-PNTL and verifying the blockage of some pores in HCP-PNTL-Co.
Figure 3. (A) Nitrogen adsorption and desorption isotherms of HCP-PNTL and HCP-PNTL-Co; (B) Pore size distribution calculated using DFT methods of HCP-PNTL and HCP-PNTL-Co. HCP: Hypercrosslinked polymer; PNTL: 1,10-phenanthroline; DFT: density functional theory.
The CO2 gas adsorption capacity of phenanthroline-based HCPs was assessed based on their high specific surface area, microporosity, and abundant nitrogen atoms [Supplementary Table 2]. Figure 4A and B shows that HCP-PNTL-A has the highest CO2 adsorption capacity of 3.2 mmol·g-1 (1 bar, 273 K) and 1.9 mmol·g-1
Catalytic performance of cycloaddition
The application of HCP-PNTL-Co in the CO2 cycloaddition reaction with epoxides was initially explored using propylene oxide (PO) as the substrate. As shown in Table 1, the reaction failed to occur in the absence of catalyst and cocatalyst (entry 1). Nevertheless, the conversion yields of PO were limited without the inclusion of catalyst (21%, Table 1, entry 2) or cocatalyst (7% or 9%, Table 1, entry 3 or entry 4), indicating that both catalyst and cocatalyst are indispensable in the cycloaddition reaction. The conversion yield of PO with HCP-PNTL-Co-A reached 75% in the presence of TBAB at 48 h, realizing a relatively efficient CO2 conversion under ambient conditions (Table 1, entry 5). In contrast, HCP-PNTL-Co-B displayed higher activity than HCP-PNTL-Co-A; a high yield of up to 95% was obtained in 48 h with the turnover number (TON) [turnover frequency (TOF)] of 5,380 (112 h-1). By comparing the activity of other catalysts in the table [Supplementary Table 3], it can be seen that HCP-PNTL-Co-B is also superior to most reported heterogeneous catalysts under mild conditions. It has significant advantages in TON (5,380) compared to some catalysts with high TON values, such as 2,2’-bipyridine zinc(II)-based hierarchical meso/microporous polymers (Bp-Zn@MA) (3,378)[56]; COF-salen-Co (3,744)[57]; P-POF-Zn (33,323)[58], etc. Although the specific surface area of HCP-PNTL-Co-B is smaller than that of HCP-PNTL-Co-A, HCP-PNTL-Co-B has a higher nitrogen content. Considering the small difference in the adsorption capacity of CO2 and Co2+ content between HCP-PNTL-Co-A and HCP-PNTL-Co-B, the higher catalytic performance of HCP-PNTL-Co-B may be due to the stronger adsorption of CO2 by HCP-PNTL-Co-B with higher nitrogen content, which concentrates CO2 around the catalytic sites[46-49]. This process not only increases the concentration of CO2 but also activates the inert CO2 molecules adsorbed on the surface, thereby improving the conversion efficiency[59]. It can more effectively activate the adsorbed CO2 molecules to excited states, making them easier to convert. At the same time, the above results also reveal that the effective activation of CO2 molecules is crucial for improving the efficiency of CO2 catalytic conversion. The possible mechanism of the HCP-PNTL-Co catalyzed CO2 cycloaddition is shown in Supplementary Figure 6.
Catalytic coupling of CO2 and PO using various catalytic systemsa
![]() | ||||||
Entry | Catalyst | TBAB | Substrate | Time (h) | Yieldb (%) | TON (TOF·h-1) |
1 | None | None | PO | 48 | 0 | - |
2 | None | 1.2 mmol | PO | 48 | 21 | - |
3 | HCP-PNTL-Co-A | None | PO | 48 | 7 | 344 (7) |
4 | HCP-PNTL-Co-B | None | PO | 48 | 9 | 663 (14) |
5 | HCP-PNTL-Co-A | 1.2 mmol | PO | 48 | 75 | 3,681 (77) |
6 | HCP-PNTL-Co-B | 1.2 mmol | PO | 48 | 95 | 5,380 (112) |
Further investigations were conducted on the effects of reaction conditions, including reaction time, various cocatalysts, and the amount of cocatalyst TBAB, using HCP-PNTL-Co-B under mild conditions (0.1 MPa, 25 °C). The yield of propylene carbonate (PC) was only 39% within the first 12 h of reaction, despite the rapid conversion of PO during this period [Figure 5A]. Moreover, the yield of product gradually increased by further prolonging the reaction time, and the PC yield could reach 95% within 48 h. Subsequently, the effects of different cocatalysts on the PO conversion were investigated under the same conditions (0.1 MPa, 25 °C). Figure 5B showed that the conversion of PO was only 46% with N,N-Dimethylpyridin-4-amine (DMAP) as the cocatalyst, while the PC yield increased dramatically to 95% or 89% when TBAB or TPPB was employed as the cocatalyst. Since the highest yield was achieved when TBAB was used as a cocatalyst, it was selected as the optimal cocatalyst for the catalytic reaction. The effects of cocatalyst (TBAB) amount on the cycloaddition reactions were also evaluated using HCP-PNTL-Co-B [Figure 5C]. The yield of PC could still reach 75% when the amount of TBAB was only 0.6 mmol, illustrating the high catalytic activity of HCP-PNTL-Co-B for cycloaddition reactions. As the amount of TBAB increased, the PC yield also increased from 75% to 95%. The preceding results indicated the optimal reaction conditions for the catalytic system as follows: a reaction duration of 48 h, employing TBAB as a cocatalyst at a quantity of 1.2 mmol.
Figure 5. Influence of (A) reaction time, (B) different cocatalysts and (C) amount of TBAB on the catalytic performance of HCP-PNTL-Co-B; (D) Recyclability of HCP-PNTL-Co-B for the chemical conversion of CO2 under ambient conditions of room temperature and atmospheric pressure. TBAB: tetrabutylammonium bromide; HCP: hypercrosslinked polymer; PNTL: 1,10-phenanthroline.
Reusability is a key factor in heterogeneous catalysis; the reusability and structural stability of HCP-PNTL-Co-B were evaluated. As shown in Figure 5D, HCP-PNTL-Co-B maintained the high efficiency after six cycles, which showed good cycling stability. The structural characterization of spent HCP-PNTL-Co-B revealed identical FT-IR spectra [Supplementary Figure 7] to that of fresh HCP-PNTL-Co-B, which confirms their excellent recycling catalytic behavior. Diversifying the range of substrates with HCP-PNTL-Co-B as the catalyst, the catalytic system demonstrated effectiveness with various terminal epoxides as well [Table 2]. Various epoxides were effectively transformed into their respective cyclic carbonates, yielding high percentages of 89%-95% and high TONs of 5,040-5,380. The more inert 2, 3-epoxide propyl butyl ether (entry 6) and 1, 2-epoxide tetradecane (entry 7) can also be efficiently converted with yields of 94% and 92%, respectively. These results show that HCP-PNTL-Co-B has good universality and can catalyze different types of epoxy substrates efficiently. The products of the CO2 conversion reaction were identified by 1H NMR spectra [Supplementary Figures 8-14].
Different substituted epoxides were catalytically coupled with CO2 using HCP-PNTL-Co-B under ambient conditions of room temperature and atmospheric pressurea
Entry | Epoxides | Products | Time (h) | Yieldsb (%) | TON |
1 | ![]() | ![]() | 48 | 95 | 5,380 |
2 | ![]() | ![]() | 48 | 94 | 5,323 |
3 | ![]() | ![]() | 48 | 95 | 5,380 |
4 | ![]() | ![]() | 48 | 95 | 5,380 |
5 | ![]() | ![]() | 48 | 89 | 5,040 |
6 | ![]() | ![]() | 48 | 94 | 5,323 |
7 | ![]() | ![]() | 48 | 92 | 5,213 |
Photocatalytic performances for CO2 reduction
To investigate the photoelectric characteristics and applicability of HCP-PNTL and HCP-PNTL-Co for photocatalytic reduction of CO2, solid-state UV-vis spectroscopy measurement was performed. As illustrated in Figure 1E and F, all polymers exhibited a wide and strong visible-light absorption region up to approximately 500 nm, while the absorption edge of HCP-PNTL-Co had a slight redshift, indicating a slight enhancement of light absorption capability with the introduction of metal Co2+. The results of the UV-vis spectroscopy indicate that HCP-PNTL-Co has strong light absorption capacity, providing a guarantee for the photocatalytic CO2 reduction under visible light.
The photocatalytic performance of HCP-PNTL-Co was studied using [Ru(bpy)3]Cl2 as the photosensitizer and TEOA as the sacrificial agent in a mixed solvent of MeCN and H2O under 1 atm CO2 and visible light irradiation. Figure 6A illustrates the time-dependent yields of CO and H2 for HCP-PNTL-Co. HCP-PNTL-Co-B demonstrated the highest photocatalytic activity, with production rates of CO and H2 reaching 2,173 and 414 μmol·g-1·h-1 after three hours, respectively, resulting in a CO selectivity of 84%.The photocatalytic activity of HCP-PNTL-Co-A was slightly inferior to that of HCP-PNTL-Co-B, exhibiting lower production rates of CO and H2 of 1,726 and 491 μmol·g-1·h-1, respectively, and a CO selectivity of 78%. Given the slight variance in CO2 adsorption capability between HCP-PNTL-Co-A and HCP-PNTL-Co-B, the higher catalytic efficiency may be attributed to the stronger adsorption force between HCP-PNTL-Co-B with higher N contents and CO2, which can activate the adsorbed CO2 molecules to excited states more effectively, thereby facilitating their conversion. The results of CO2 photocatalytic reduction were consistent with the above cycloaddition reactions, indicating that HCP-PNTL-Co-A and HCP-PNTL-Co-B with abundant N heteroatoms have high CO2 photocatalytic reduction ability. Furthermore, this clearly demonstrates the potential of metal ion doping in HCPs for photocatalytic CO2 reduction and the effectiveness of introducing CO2-philic active sites to enhance CO2 catalytic conversion efficiency. The photocatalytic performance of HCP-PNTL-B modified with other metal ions such as Fe2+, Mn2+, or Ni2+ was evaluated under identical reaction conditions, and the chemical structure, thermal stability and porosity characteristics and gas adsorption capacities were performed [Supplementary Figures 15-20]. As shown in Figure 6B, HCP-PNTL-Ni-B exhibits the highest catalytic activity, producing a large amount of CO
Figure 6. The production rate of CO and H2 evolution over (A) HCP-PNTL-Co and (B) HCP-PNTL-M-B under visible-light irradiation; (C) The production rate of CO and H2 evolution under various conditions of HCP-PNTL-Ni-B and HCP-PNTL-Co-B; (D) Cyclic stability test of HCP-PNTL-Ni-B and HCP-PNTL-Co-B. HCP: Hypercrosslinked polymer; PNTL: 1,10-phenanthroline.
Control experiments were conducted to ascertain the influence of various reaction factors in the photocatalytic system [Figure 6C]. The production rate of CO was only 136 μmol·g-1·h-1 with HCP-PNTL-B as the catalyst for photocatalytic CO2 reduction, and the selectivity for CO was only 36.8%, indicating that metal Ni2+ or Co2+ is the catalytic active site for photocatalytic CO2 reduction. Under the condition of using metal Ni2+ or Co2+ alone as the catalyst, the production rate and selectivity of CO was only 683 μmol·g-1·h-1 and 78% or 533 μmol·g-1·h-1 and 73%, much lower than that of HCP-PNTL-Ni-B or HCP-PNTL-Co-B, indicating that the existence of HCP-PNTL-B in the catalytic system improved the CO2 adsorption and activation ability and the stable dispersion of Ni2+ or Co2+ catalytic sites, which can significantly enhance the photocatalytic CO2 reduction performance. When the photosensitizer was absent from the reaction system, only trace amounts of CO and H2 were detectable, while negligible amounts were obtained without a sacrificial agent or light irradiation during the photocatalytic reduction reaction. Substituting N2 for CO2 in the photocatalytic reaction resulted in a significant amount of H2 (492 μmol·g-1·h-1) but no detectable CO. The control experiments demonstrated the essential nature of all the previously mentioned reaction factors for the photocatalytic conversion of CO2. Lastly, the impact of solvents on the photocatalytic CO2 reduction performance of HCP-PNTL-Ni-B was examined. As shown in Supplementary Figure 21, HCP-PNTL-Ni-B exhibited the optimal photocatalytic activity in MeCN. The higher photocatalytic performance may be that, compared to other solvents, MeCN has a higher solubility for CO2, which is conducive to the increase of CO2 concentration near the catalytic sites. Similar reaction mechanisms for the catalytic reduction of CO2 by metal-based POPs photocatalytic systems under catalytic conditions have been reported in related studies. Based on literature reports[64,65] and control experimental results, the possible mechanism of
To confirm the source of CO, isotope-labeled 13CO2 was utilized as the substrate for the photocatalytic reaction under identical conditions, and the resulting product was identified using gas chromatography-mass spectrometry (GC-MS). As shown in Supplementary Figure 22, the presence of a peak at m/z = 29 indicated that the generated 13CO originated from gaseous 13CO2 species rather than the decomposition of other organic compounds, such as the photocatalyst or solvent, in the photocatalytic system. It can be seen from Figure 6D that there was no obvious loss of the photocatalytic activity of HCP-PNTL-Ni-B or HCP-PNTL-Co-B after four-run cycling experiments, verifying its superior reusability in the photocatalytic reaction. Furthermore, negligible changes were observed in the FT-IR spectra [Supplementary Figure 23] of HCP-PNTL-Ni-B before and after the photocatalytic cycling reactions, confirming the structural stability and photocatalytic durability of HCP-PNTL-Ni-B in the photocatalytic CO2 reduction process.
CONCLUSIONS
In summary, PNTL derivatives were selected as functional monomers for the HCP synthesis via the Friedel-Crafts reaction. Subsequently, a composite catalytic system was devised by incorporating Co2+ for use in the cycloaddition reaction of CO2 with epoxides and the photoreduction of CO2 under ambient conditions. The nitrogen content in the structure of HCPs was enhanced by directly regulating the structure of the functional monomer to increase the CO2 adsorption capacity and activation ability, which can avoid the uneven distribution of CO2-philic active sites introduced by post-functionalization. The composite catalytic system with the highest nitrogen content (5.6 wt%), HCP-PNTL-Co-B, achieved CO2 adsorption capacity and heat of adsorption of 2.0 mmol/g (273 K, 1 bar) and 33.0 kJ·mol-1, respectively. In the presence of cocatalyst TBAB, HCP-PNTL-Co-B also showed higher catalytic activity, achieving efficient catalysis of the cycloaddition reaction of CO2 with epoxides under ambient pressure and temperature with a high yield of 95% after 48 h and a TON (TOF) value of up to 5,380 (112 h-1). Meanwhile, HCP-PNTL-Co-B also achieved a high CO production rate of 2,173 μmol·g-1·h-1 and a high selectivity of 84% in the photoreduction reaction. Moreover, the composite catalytic system exhibited good structural stability in the cycling experiments. The study has shown that introducing CO2-philic active sites improves the CO2 adsorption and activation capacity of materials, offering a novel research strategy for developing and designing efficient CO2 catalytic systems. Future research efforts can be conducted in-depth and systematically around (1) enhancing the conversion efficiency of materials for CO2 under mild conditions by adjusting the microstructure of catalysts or optimizing reaction conditions; (2) improving the structural stability of materials to ensure their efficient performance during cycling; (3) elucidating the catalytic mechanism and establishing the structure-performance relationship between polymer structure and catalytic properties.
DECLARATIONS
Acknowledgments
The authors thank the Analysis and Testing Center at Huazhong University of Science and Technology for their assistance in the characterization of materials.
Authors’ contributions
Made substantial contributions to conception and design of the study and performed data analysis and interpretation: Ouyang H, Peng M
Performed data acquisition and provided administrative, technical, and material support: Song K, Wang S, Gao H, Tan B
Availability of data and materials
The authors confirm that the data supporting the findings of this study are available within its Supplementary Materials.
Financial support and sponsorship
This work was financially supported by the National Natural Science Foundation of China (Grant Nos. 22161142005, 21975086), the International S&T Cooperation Program of China (Grant No. 2018YFE0117300), Science and Technology Department of Hubei Province (No. 2019CFA008), and the Open Research Fund (No. 2023JYBKF04) of Key Laboratory of Material Chemistry for Energy Conversion and Storage (HUST), Ministry of Education.
Conflicts of interest
All authors declared that there are no conflicts of interest.
Ethical approval and consent to participate
Not applicable.
Consent for publication
Not applicable.
Copyright
© The Author(s) 2024.
Supplementary Materials
REFERENCES
1. Ji G, Zhao Y, Liu Z. Design of porous organic polymer catalysts for transformation of carbon dioxide. Green Chem Eng. 2022;3:96-110.
2. Truong CC, Mishra DK. Catalyst-free fixation of carbon dioxide into value-added chemicals: a review. Environ Chem Lett. 2021;19:911-40.
3. Cheng Y, Ding X, Han B. Porous organic polymers for photocatalytic carbon dioxide reduction. ChemPhotoChem. 2021;5:406-17.
4. Zhang XY, Wang P, Zhang Y, Cheng XM, Sun WY. Facet-dependent photocatalytic behavior of Fe-soc-MOF for carbon dioxide reduction. ACS Appl Mater Interfaces. 2023;15:3348-56.
5. Steinlechner C, Junge H. Renewable methane generation from carbon dioxide and sunlight. Angew Chem Int Ed Engl. 2018;57:44-5.
6. Liao X, Wang Z, Kong L, et al. Synergistic catalysis of hypercrosslinked ionic polymers with multi-ionic sites for conversion of CO2 to cyclic carbonates. Mol Catal. 2023;535:112834.
7. Li PZ, Wang XJ, Liu J, Lim JS, Zou R, Zhao Y. A triazole-containing metal-organic framework as a highly effective and substrate size-dependent catalyst for CO2 conversion. J Am Chem Soc. 2016;138:2142-5.
8. Truong CC, Mishra DK. Recent advances in the catalytic fixation of carbon dioxide to value-added chemicals over alkali metal salts. J CO2 Util. 2020;41:101252.
9. Zhao Y, Han B, Liu Z. Ionic-liquid-catalyzed approaches under metal-free conditions. Acc Chem Res. 2021;54:3172-90.
10. Chen Y, Mu T. Conversion of CO2 to value-added products mediated by ionic liquids. Green Chem. 2019;21:2544-74.
11. Aomchad V, Del Gobbo S, Yingcharoen P, Poater A, D’elia V. Exploring the potential of group III salen complexes for the conversion of CO2 under ambient conditions. Catal Today. 2021;375:324-34.
12. Lan D, Gong Y, Tan N, et al. Multi-functionalization of GO with multi-cationic ILs as high efficient metal-free catalyst for CO2 cycloaddition under mild conditions. Carbon. 2018;127:245-54.
13. Cui X, Dai X, Surkus A, et al. Zinc single atoms on N-doped carbon: an efficient and stable catalyst for CO2 fixation and conversion. Chinese J Catal. 2019;40:1679-85.
14. Jayakumar S, Li H, Tao L, et al. Cationic Zn-porphyrin immobilized in mesoporous silicas as bifunctional catalyst for CO2 cycloaddition reaction under cocatalyst free conditions. ACS Sustain Chem Eng. 2018;6:9237-45.
15. Miralda CM, Macias EE, Zhu M, Ratnasamy P, Carreon MA. Zeolitic imidazole framework-8 catalysts in the conversion of CO2 to chloropropene carbonate. ACS Catal. 2012;2:180-3.
16. Zhu M, Srinivas D, Bhogeswararao S, Ratnasamy P, Carreon MA. Catalytic activity of ZIF-8 in the synthesis of styrene carbonate from CO2 and styrene oxide. Catal Commun. 2013;32:36-40.
17. Ding M, Jiang H. Incorporation of imidazolium-based poly(ionic liquid)s into a metal-organic framework for CO2 capture and conversion. ACS Catal. 2018;8:3194-201.
18. Sun Q, Aguila B, Perman J, Nguyen N, Ma S. Flexibility matters: cooperative active sites in covalent organic framework and threaded ionic polymer. J Am Chem Soc. 2016;138:15790-6.
19. Zhi Y, Shao P, Feng X, et al. Covalent organic frameworks: efficient, metal-free, heterogeneous organocatalysts for chemical fixation of CO2 under mild conditions. J Mater Chem A. 2018;6:374-82.
20. Sun Q, Dai Z, Meng X, Xiao FS. Porous polymer catalysts with hierarchical structures. Chem Soc Rev. 2015;44:6018-34.
21. Zhang Y, Riduan SN. Functional porous organic polymers for heterogeneous catalysis. Chem Soc Rev. 2012;41:2083-94.
22. Ma D, Li J, Liu K, Li B, Li C, Shi Z. Di-ionic multifunctional porous organic frameworks for efficient CO2 fixation under mild and co-catalyst free conditions. Green Chem. 2018;20:5285-91.
23. Liu J, Zhao G, Cheung O, Jia L, Sun Z, Zhang S. Highly porous metalloporphyrin covalent ionic frameworks with well-defined cooperative functional groups as excellent catalysts for CO2 cycloaddition. Chemistry. 2019;25:9052-9.
24. Bhanja P, Modak A, Bhaumik A. Porous organic polymers for CO2 storage and conversion reactions. ChemCatChem. 2019;11:244-57.
25. Li B, Gong R, Wang W, et al. A new strategy to microporous polymers: knitting rigid aromatic building blocks by external cross-linker. Macromolecules. 2011;44:2410-4.
26. Gu Y, Son SU, Li T, Tan B. Low-cost hypercrosslinked polymers by direct knitting strategy for catalytic applications. Adv Funct Mater. 2021;31:2008265.
27. Tan L, Tan B. Functionalized hierarchical porous polymeric monoliths as versatile platforms to support uniform and ultrafine metal nanoparticles for heterogeneous catalysis. Chem Eng J. 2020;390:124485.
28. Gao TN, Wang T, Wu W, et al. Solvent-induced self-assembly strategy to synthesize well-defined hierarchically porous polymers. Adv Mater. 2019;31:1806254.
29. Wang S, Zhang C, Shu Y, et al. Layered microporous polymers by solvent knitting method. Sci Adv. 2017;3:e1602610.
30. Li J, Han Y, Ji T, et al. Porous metallosalen hypercrosslinked ionic polymers for cooperative CO2 cycloaddition conversion. Ind Eng Chem Res. 2020;59:676-84.
31. Liao X, Wang Z, Li Z, et al. Tailoring hypercrosslinked ionic polymers with high ionic density for rapid conversion of CO2 into cyclic carbonates at low pressure. Chem Eng J. 2023;471:144455.
32. Wang S, Song K, Zhang C, Shu Y, Li T, Tan B. A novel metalporphyrin-based microporous organic polymer with high CO2 uptake and efficient chemical conversion of CO2 under ambient conditions. J Mater Chem A. 2017;5:1509-15.
33. Xu W, Chen M, Yang Y, et al. Construction of aluminum-porphyrin-based hypercrosslinked ionic polymers (HIPs) by direct knitting approach for CO2 capture and in-situ conversion to cyclic carbonates. ChemCatChem. 2023;15:e202201441.
34. Kunitski M, Eicke N, Huber P, et al. Double-slit photoelectron interference in strong-field ionization of the neon dimer. Nat Commun. 2019;10:1.
35. Zhan Z, Wang H, Huang Q, et al. Grafting hypercrosslinked polymers on TiO2 surface for anchoring ultrafine Pd nanoparticles: dramatically enhanced efficiency and selectivity toward photocatalytic reduction of CO2 to CH4. Small. 2022;18:e2105083.
36. Manigrasso J, Chillón I, Genna V, et al. Author correction: visualizing group II intron dynamics between the first and second steps of splicing. Nat Commun. 2022;13:1.
37. Chen Y, Luo R, Xu Q, Zhang W, Zhou X, Ji H. State-of-the-art aluminum porphyrin-based heterogeneous catalysts for the chemical fixation of CO2 into cyclic carbonates at ambient conditions. ChemCatChem. 2017;9:767-73.
38. Yuan YC, Mellah M, Schulz E, David ORP. Making chiral salen complexes work with organocatalysts. Chem Rev. 2022;122:8841-83.
39. Liao X, Xiang X, Wang Z, et al. A novel crosslinker for synthesizing hypercrosslinked ionic polymers containing activating groups as efficient catalysts for the CO2 cycloaddition reaction. Sustain Energy Fuels. 2022;6:2846-57.
40. Li HB, Yu MH, Wang FX, et al. Amorphous nickel hydroxide nanospheres with ultrahigh capacitance and energy density as electrochemical pseudocapacitor materials. Nat Commun. 2013;4:1894.
41. Li P, Wang X, Liu J, Phang HS, Li Y, Zhao Y. Highly effective carbon fixation via catalytic conversion of CO2 by an acylamide-containing metal-organic framework. Chem Mater. 2017;29:9256-61.
42. Dai Z, Sun Q, Liu X, et al. Metalated porous porphyrin polymers as efficient heterogeneous catalysts for cycloaddition of epoxides with CO2 under ambient conditions. J Catal. 2016;338:202-9.
43. Li J, Han Y, Lin H, et al. Cobalt-salen-based porous ionic polymer: the role of valence on cooperative conversion of CO2 to cyclic carbonate. ACS Appl Mater Interfaces. 2020;12:609-18.
44. Xie Y, Liang J, Fu Y, et al. Hypercrosslinked mesoporous poly(ionic liquid)s with high ionic density for efficient CO2 capture and conversion into cyclic carbonates. J Mater Chem A. 2018;6:6660-6.
45. Ma Y, Tang Q, Sun W, et al. Assembling ultrafine TiO2 nanoparticles on UiO-66 octahedrons to promote selective photocatalytic conversion of CO2 to CH4 at a low concentration. Appl Catal B Environ. 2020;270:118856.
46. Liu M, Shao L, Huang J, Liu Y. O-containing hyper-cross-linked polymers and porous carbons for CO2 capture. Micropor Mesopor Mater. 2018;264:104-11.
47. Liao C, Liang Z, Liu B, Chen H, Wang X, Li H. Phenylamino-, phenoxy-, and benzenesulfenyl-linked covalent triazine frameworks for CO2 capture. ACS Appl Nano Mater. 2020;3:2889-98.
48. Shao L, Sang Y, Liu N, et al. Selectable microporous carbons derived from poplar wood by three preparation routes for CO2 capture. ACS Omega. 2020;5:17450-62.
49. Shao L, Liu M, Sang Y, Zhan P, Chen J, Huang J. Nitrogen-doped ultrahigh microporous carbons derived from two nitrogen-containing post-cross-linked polymers for efficient CO2 capture. J Chem Eng Data. 2020;65:2238-50.
50. Yang K, Yang Z, Zhang C, et al. Recent advances in CdS-based photocatalysts for CO2 photocatalytic conversion. Chem Eng J. 2021;418:129344.
51. Shen Y, Zheng Q, Zhu H, Tu T. Hierarchical porous organometallic polymers fabricated by direct knitting: recyclable single-site catalysts with enhanced activity. Adv Mater. 2020;32:e1905950.
52. Wen D, Zheng Q, Yang S, Zhu H, Tu T. Direct knitting boosts the stability and catalytic activity of NHC-Au complexes towards valorization of SO2 and CO2. J Catal. 2023;418:64-9.
53. Nunes P, Correia I, Marques F, et al. Copper complexes with 1,10-phenanthroline derivatives: underlying factors affecting their cytotoxicity. Inorg Chem. 2020;59:9116-34.
54. Pu Y, Harding RE, Stevenson SG, et al. Solution processable phosphorescent rhenium(i) dendrimers. J Mater Chem. 2007;17:4255-64.
55. Ge Z, Hayakawa T, Ando S, et al. Synthesis and properties of 3,8-Bis[4-(9H-carbazol-9-yl)phenyl]-1,10-phenanthroline for phosphorescent OLEDs. Chem Lett. 2008;37:262-3.
56. Chen J, Li H, Zhong M, Yang Q. Hierarchical mesoporous organic polymer with an intercalated metal complex for the efficient synthesis of cyclic carbonates from flue gas. Green Chem. 2016;18:6493-500.
57. Li H, Feng X, Shao P, et al. Synthesis of covalent organic frameworks via in situ salen skeleton formation for catalytic applications. J Mater Chem A. 2019;7:5482-92.
58. Chen J, Zhong M, Tao L, et al. The cooperation of porphyrin-based porous polymer and thermal-responsive ionic liquid for efficient CO2 cycloaddition reaction. Green Chem. 2018;20:903-11.
59. Ouyang H, Song K, Du J, Zhan Z, Tan B. Creating chemisorption sites for enhanced CO2 chemical conversion activity through amine modification of metalloporphyrin-based hypercrosslinked polymers. Chem Eng J. 2022;431:134326.
60. Dong Y, Liu H, Wang S, Guan G, Yang Q. Immobilizing isatin-schiff base complexes in NH2 -UiO-66 for highly photocatalytic CO2 reduction. ACS Catal. 2023;13:2547-54.
61. Cui J, Fu Y, Meng B, et al. A novel cobalt-anchored covalent organic framework for photocatalytic conversion of CO2 into widely adjustable syngas. J Mater Chem A. 2022;10:13418-27.
62. Yang Y, Lu Y, Zhang H, et al. Decoration of active sites in covalent-organic framework: an effective strategy of building efficient photocatalysis for CO2 reduction. ACS Sustain Chem Eng. 2021;9:13376-84.
63. Zhong W, Sa R, Li L, et al. A covalent organic framework bearing single Ni sites as a synergistic photocatalyst for selective photoreduction of CO2 to CO. J Am Chem Soc. 2019;141:7615-21.
64. Han B, Ou X, Zhong Z, Liang S, Deng H, Lin Z. Rational design of FeNi bimetal modified covalent organic frameworks for photoconversion of anthropogenic CO2 into widely tunable syngas. Small. 2020;16:e2002985.
Cite This Article

How to Cite
Download Citation
Export Citation File:
Type of Import
Tips on Downloading Citation
Citation Manager File Format
Type of Import
Direct Import: When the Direct Import option is selected (the default state), a dialogue box will give you the option to Save or Open the downloaded citation data. Choosing Open will either launch your citation manager or give you a choice of applications with which to use the metadata. The Save option saves the file locally for later use.
Indirect Import: When the Indirect Import option is selected, the metadata is displayed and may be copied and pasted as needed.
About This Article
Special Issue
Copyright
Author Biographies
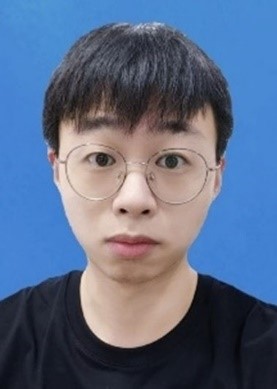
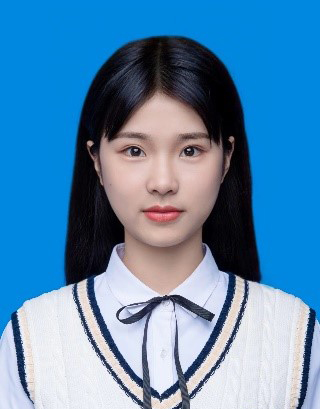
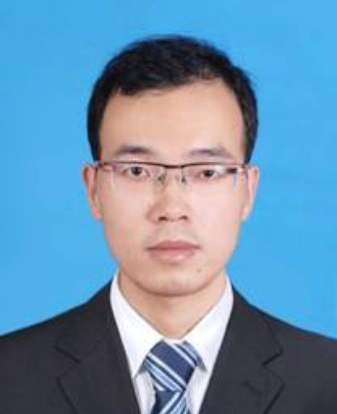
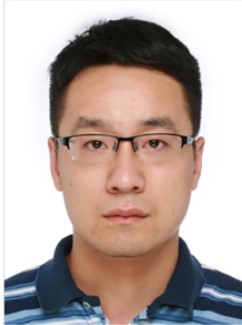
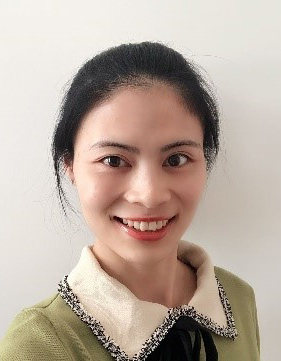
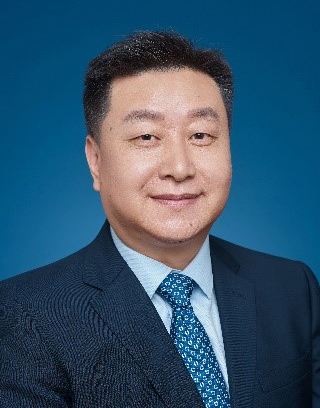
Data & Comments
Data
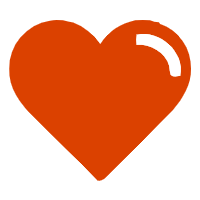
Comments
Comments must be written in English. Spam, offensive content, impersonation, and private information will not be permitted. If any comment is reported and identified as inappropriate content by OAE staff, the comment will be removed without notice. If you have any queries or need any help, please contact us at support@oaepublish.com.