Age-related hearing loss: possible associations with Alzheimer’s disease
Abstract
With the increasing aging of the population, there has been a growing focus on the association between age-related hearing loss (ARHL) and Alzheimer’s disease (AD). Given the extended latency period of AD, it has been assumed that mechanisms contributing to its pathogenesis may also contribute to ARHL. Abnormal deposition of beta-amyloid (Aβ) and tau protein in the brain serves as the primary etiological marker for AD, being produced long before the symptomatic onset of AD. The neurotoxicity induced by their unique oligomers not only exerts significant effects on the central nervous system but also exhibits similar impacts on certain peripheral organs. In this review, we analyze the factors leading to β-amyloid and tau production, explore their cascading effects and roles in AD progression, discuss the possible influence of auditory deprivation on cognitive functions, and investigate the potential causes of hearing loss by examining other peripheral organs in AD patients and model animals as examples. These findings provide further evidence supporting ARHL as an early indicator of AD.
Keywords
INTRODUCTION
Age-related hearing loss
Age-related hearing loss (ARHL), also known as presbyacusis, is a progressive, bilateral, and symmetrical sensorineural hearing loss caused by the aging auditory system[1]. It is characterized by a decline in auditory sensitivity and impaired speech perception. ARHL can be attributed to various risk factors such as noise exposure[2], smoking[3], medications[4], hypertension, family history, etc. [Figure 1]. Multiple structures of the cochlea, including the stria vascularis and its vasculature, spiral ligaments, sensory hair cells, and auditory neurons, may be affected[5]. With global population growth and an aging population worldwide, there has been a rapid increase in the prevalence of ARHL. According to a report from the World Health Organization, approximately 466 million people worldwide are currently experiencing varying degrees of hearing loss. Without intervention or action taken on this issue, this number will rise to 630 million by 2030 and exceed 900 million by 2050. Extensive evidence demonstrates that ARHL negatively impacts physical health, mental well-being, cognitive function, independence, social interactions, and overall quality of life among older adults[6]. Furthermore, the untreated condition imposes significant social and economic burdens globally, with an estimated annual loss of US $750 billion[7]. The substantial number of individuals affected has made hearing loss a pressing public health concern on a global scale. Currently, the most common treatment for ARHL involves the use of hearing aids[8], which not only improves overall elderly health conditions but also serves as a more cost-effective rehabilitation strategy compared to cochlear implants (CI). However, the effectiveness of hearing aids is heavily dependent on the remaining functional hair cells. More promising strategies for hearing loss, such as gene therapy, are in high demand.
Figure 1. The risk factors of ARHL and AD. ARHL and AD are both complex conditions related to many risk factors. Previous epidemiological studies have indicated there are certain links between ARHL and AD. However, the underlying mechanisms linking these two diseases remain elusive. ARHL: Age-related hearing loss; AD: Alzheimer’s disease.
Alzheimer’s disease
Alzheimer’s disease (AD) is the most prevalent form of dementia, accounting for 60% to 80% of all cases[9]. AD can be broadly categorized into two forms: early-onset/familial AD and late-onset/sporadic AD. Familial AD is caused by mutations in the genes encoding β-amyloid precursor protein (APP), presenilin 1 (PSEN1), and presenilin 2 (PSEN2). The onset of familial AD typically occurs before the age of 65 years, with a remarkably low prevalence rate, constituting only 1%-2% of all AD cases[10,11]. However, late-onset AD may involve various factors such as unhealthy lifestyle choices, traumatic brain injury (TBI), diabetes, diverse cardiovascular diseases, kidney diseases, epigenetic factors, and environmental and occupational exposures [Figure 1][12,13]. As a progressive neurodegenerative disorder, brain alterations in individuals with AD may commence more than two decades prior to symptom onset[14]. Over time, neuronal damage worsens and affects multiple regions of the brain, leading to memory loss, including severe cognitive dysfunction in later stages, along with impairment in language skills and thinking abilities, ultimately resulting in loss of self-care capacity. This triggers a cascade of complications, culminating in death. As per estimates from 2018, approximately 50 million people worldwide are affected by this disease[15], which is projected to rise exponentially due to an aging population reaching around 75 million patients by 2030[16]. It has been over a century since its discovery as a fatal condition named AD. However, the intricate molecular mechanisms underlying its pathophysiology remain incompletely elucidated, and an effective cure is still elusive. Given its challenging diagnosis at later stages, coupled with its refractory nature and high mortality rate, prevention strategies and interventions aimed at slowing down AD continue to be our utmost priority.
Epidemiological association between ARHL and AD
Since the initial report by Kay et al. in 1964, a growing number of epidemiological evidence from case-control, cross-sectional, and longitudinal population-based studies has consistently demonstrated an independent link between hearing loss and impairment in cognitive functions[17]. Furthermore, these studies have shown that hearing loss is associated with accelerated cognitive decline and a higher incidence of dementia[18,19]. In a seven-year follow-up study involving approximately 16,000 Hispanic and Latino adults[20], hearing loss was found to be associated with greater cognitive decline as well as slower changes in processing speed after seven years. Cantuaria et al. investigated the relationship between hearing levels and dementia using personal hearing status data recorded in the Southern Danish Hearing Examination database[21]. Their findings revealed that severe hearing levels were significantly associated with an increased risk of dementia compared to individuals without hearing loss. The calculated hazard ratios (HRs) were 1.20 (95%CI, 1.09-1.32) for those with mild-to-moderate hearing loss and 1.13 (95%CI, 1.06-1.20) for those without any use of hearing aids, suggesting that the progression of dementia may be somewhat delayed by the use of such devices among individuals experiencing ARHL symptoms. Lin et al. also suggested that early intervention for improving auditory function may potentially reduce cognitive changes within three years among individuals aged over 70 who are at an elevated risk for cognitive decline[22]. Moreover, Kwok et al. investigated the association between ARHL and AD by conducting pure tone audiometry on AD patients[23]. They discovered a significant increase in the hearing threshold of pure tone audiometry in AD patients compared to the control group. The pure-tone averages calculated from air conduction thresholds at 500, 1,000, and 2,000 Hz (0.5-2 kHz PTA) showed a 2.3 dB HL elevation in AD subjects compared to controls (P = 0.001). Numerous studies have demonstrated a close relationship between ARHL and AD. On one hand, cognitive decline leads to impaired auditory system function in AD patients. On the other hand, hearing loss may act as a risk factor for dementia and AD development. However, it remains unclear whether hearing loss causes AD or if it is an early manifestation of the condition[24]. Considering the long latency period of AD, this review proposes that ARHL could be an early symptom of long-term pathological accumulation leading to AD pathology. Thus, it is possible to utilize hearing loss as an early marker to prevent AD progression. Furthermore, we aim to elucidate the etiology of ARHL by understanding the pathogenic mechanisms underlying AD itself [Figure 1].
CATEGORY AND PATHOLOGY OF ARHL
Categories of ARHL
ARHL (presbycusis) refers to bilaterally symmetrical hearing loss resulting from the aging process. ARHL is commonly classified into four categories based on the results of audiometric tests and temporal bone pathology as established by Schuknecht (1969)[25]:
(1) Sensory: this type of ARHL stems from the degenerating organ of Corti and is primarily caused by damaged outer hair cells, evidenced in both human[25-27] and animal studies[28-30]. Patients experience hearing loss in the high-frequency range.
(2) Neural: this type of ARHL shows a loss of 50% or more of a total of 35,500 cochlear neurons. Patients have a moderate downward slope of pure tone threshold toward high-frequency and also have difficulties in speech discrimination[31,32].
(3) Metabolic or strial: this type of ARHL is caused by the atrophy of the stria vascularis. In most cases, there is a loss of 30% or more in the stria vascularis[33]. Patients show hearing loss across all frequency ranges in the audiogram.
(4) Cochlear conductive: this type of ARHL has no significant pathological changes in the cochlea, and there is no or few hair cell loss in the basal segment of the cochlea. The auditory loss may be caused by the hardening of the basement membrane or impaired motor function of the cochlear canal. Patients experience low-frequency hearing loss with unimpaired speech recognition[34].
In 1993, mixed and indeterminate types were added for a total of six categories[26].
(5) Mixed: this type of ARHL refers to a combination of the four types of hearing loss mentioned above. Patients have no specific audiometric pattern.
(6) Indeterminate: this type of ARHL has no correlation between audiometric patterns and age-related pathologic alterations in the cochlea[25,27,35].
Pathogenesis of ARHL
The causes of ARHL are considered to involve the interaction of many factors, including aging, oxidative damage, genetic factors, and environmental factors [Figure 1][36]. One of the most prevalent explanations for aging is an increase in oxygen free radicals. The cochlea contains many metabolically active tissues, which require a large amount of adenosine triphosphate (ATP) for sound conversion and maintenance of internal cochlear electrical potential. During high-energy metabolism, a large number of reactive oxygen species (ROS), also known as free radicals, are produced[37,38]. Oxidative damage caused by ROS is thought to play an important role in the pathogenesis of ARHL[39-42]. As age increases, the production of ROS also rises. Excessive ROS production can impair the intracellular antioxidant system and damage mitochondria, which then cause cytotoxic reactions and cell damage[43-45]. Mitochondria are primary targets of free radicals, and the accumulation of ROS leads to increased mutations and deletions of mitochondrial DNA (mtDNA)[46], which is considered an important factor leading to ARHL.
Studies have shown that the occurrence of age-related diseases is also closely related to chronic inflammation[47]. Various age-related diseases, such as neurodegenerative diseases, diabetes, osteoporosis, etc., are involved in inflammatory mechanisms. The cochlea is not an immune-privileged organ. Systemic inflammation contributes to presbycusis, and changes in the morphology and number of macrophages can occur in the aging cochlea. Adams[48] has found the mRNA expression of some inflammation-related factors, such as IL-6, TNF-α, NF-κB, and p65 in the cochlea.
In addition, many candidate genes have been found to be associated with ARHL by genome-wide association study (GWAS)[49]. These genes may play important roles in signaling and maintenance of the cochlear microenvironment, including glutamate metabotropic receptor 7 (GRM7)[50], or detoxification enzymes, such as uncoupling protein 2 (UCP2)[51], superoxide dismutase 2 (SOD2), N-acetyltransferase 2 (NAT2)[52], monogenic deafness-causing gene (TMC1)[53] and doublecortin-like kinase 1 (DCLK1)[54].
PATHOGENESIS OF AD
Pathogenic deposition of β-amyloid
AD is a complex disease comprising multiple pathogenic factors. Its fundamental neuropathological changes and main features include the deposition of beta-amyloid (Aβ), hyperphosphorylated tau protein, and neurofibrillary tangles (NFT), accompanied by synaptic and neuronal loss[55]. Among these, the accumulation of Aβ in the brain is an early event that plays a key role in the pathogenesis of AD[56]. It is believed that Aβ begins to accumulate 15-20 years before the onset of clinical symptoms of AD. Therefore, the concentration of Aβ42 in cerebrospinal fluid (CSF) is recognized as a useful biomarker for early diagnosis of AD[57-59]. Aβ precursor protein (APP) undergoes cleavage by β-secretase and α-secretase to produce APP C-terminal fragments (APP-CTFs) consisting of 99 amino acids (C99) and 83 amino acids (C83), respectively. Subsequently, it is cleaved by γ-secretase to generate Aβ[60]. With aging, there is an imbalance between the generation and clearance of Aβ, leading to a change in its secondary structure from a healthy α-helix to a β-sheet-enriched structure where Aβ aggregates together, forming soluble toxic oligomers[61]. Pathogenic amyloid plaques start depositing in the entorhinal cortex and gradually spread to other regions such as the hippocampus, temporal cortex, frontoparietal cortex, and subcortical nuclei[62]. Studies have revealed that Aβ can activate microglia, which trigger proinflammatory cytokine release, resulting in neuroinflammation. Conversely, released cytokines can induce APP production, which leads to more production of Aβ[55,63,64].
Aβ deposition and mitochondrial dysfunction
Neurons affected by AD initially undergo mitochondrial dysfunction and abnormal energy metabolism, thereby promoting the development of Aβ and tau pathology associated with the disease[65-67]. In turn, the continuous deposition of Aβ exacerbates mitochondrial damage and further advances disease progression[60,68]. Mitochondria are crucial organelles that regulate intracellular metabolic pathways, including ATP production through electron transport and oxidative phosphorylation (OXPHOS), tricarboxylic acid (TCA) cycle, fatty acid oxidation, amino acid synthesis, calcium homeostasis, and iron metabolism[69]. Alongside mitochondrial dysfunction in AD brains, various pathophysiological events such as apoptosis, inflammation, oxidative stress, and impaired glucose metabolism occur at the same time[70,71]. Studies have observed that diabetic rats with significant Aβ deposits exhibit reduced calorie intake, activity levels, and fat oxidation while experiencing increased carbohydrate oxidation and energy expenditure, leading to weight loss[72]. Additionally, in some studies, it is noted that there is an elevated lactate production indicated by higher lactate/pyruvate ratios along with decreased succinate/fumarate/glutamine concentrations measured in CSF from AD patients. These findings suggest mitochondrial glucose metabolism is impaired due to blood-brain barrier breakdown[73].
Mitochondria serve as the primary source of intracellular ROS, with approximately 90% of ROS originating from mitochondria[74,75]. The generated mitochondrial ROS (mtROS) exacerbates the disruption in mitochondrial energy metabolism by impairing the electron transport chain (ETC) and increasing mitochondrial outer membrane permeability (MOMP)[76]. ETC is situated within the inner mitochondrial membrane and comprises four enzyme complexes: nicotinamide adenine dinucleotide ubiquinone reductase (complex I), succinate ubiquinone oxidoreductase (complex II), ubiquinone cytochrome oxidoreductase (complex III), and cytochrome c oxidase (complex IV). Additionally, two electron carriers, ubiquinone (CoQ) and cytochrome c (Cyt C), are involved[77]. Complexes I and III represent major sites for ROS production[78,79]. Studies have revealed that AD patients commonly exhibit ETC damage, which correlates with reduced intracellular ATP levels and increased oxidative stress[80]. Dysfunction in mitochondrial ETC and OXPHOS has been associated with a direct impact of Aβ on mitochondria[81]. Moreover, mtROS can activate inflammatory pathways such as Toll-like receptors (TLRs) and NLRP3 inflammasomes to promote inflammation and harm to organs[82,83]. Due to its lack of histones and limited DNA repair capacity, mtDNA becomes vulnerable to oxidative stress. Notably, the copy number of mtDNA undergoes constant changes during aging and disease progression[84]. Clinical investigations have demonstrated lower levels of mtDNA in the frontal cortex, hippocampus, and CSF among AD patients compared to controls[85-87].
Tau and neurotoxicity
Tau protein is strongly associated with the progression of cognitive impairment. Studies have shown that with aging, tau pathology accumulates in the entorhinal cortex and the medial temporal lobe, so-called primary age-related tau lesions (PART), even in the absence of cognitive decline[88]. Tau protein plays an important role in microtubule assembly and stabilization of neuronal axons, as well as regulation of microtubule transport. Knockout (KO) mice, while not exhibiting a severe developmental phenotype, show significant delays in neuronal maturation and impaired synaptic plasticity in cell culture[89].
Under normal circumstances, tau protein is usually present in its natural monomer form, but in the brains of Alzheimer’s patients, tau protein aggregates occur in a fixed way along neuroanatomical connections. Misfolded tau proteins promote misfolding of natural tau monomers, further leading to the production of new pathological tau protein aggregates[90].
Tau protein is also affected by a variety of post-translational modifications, including phosphorylation, acetylation, glycation, O-GlcNAcylation, and other modifications[91]. Tau phosphorylation at different sites reflects the disease process. In the early stage of AD, NFTs have not formed yet, and tau phosphorylation sites mainly occur at Ser199 and Ser422. Subsequently, phosphorylation at Ser202 and Thr205 will continue to increase with the progression of the disease. Phosphorylation at Thr231 generally signals more mature p-Tau assembly to form NFTs, driving the disease to an advanced stage[90]. Acetylation also participates in this process. Recent research has demonstrated that TBI, a non-hereditary, non-aging-related risk factor in AD, induces tau acetylation (ac-tau) at the acetylation site in the brain of AD patients with a history of TBI[92].
Genetic risk factor: ApoE
Apolipoprotein E (ApoE) ε4 gene is currently the only recognized risk gene for AD. The frequency of carrying APOEε4 allele in sporadic AD patients is 40%, and the risk of AD in the population carrying one APOEε4 allele is 3-4 times that of the non-APOEε4 population. People with two APOEε4 alleles have a 12 times higher risk of developing AD than those without APOEε4[93]. ApoE is involved in the production of
POSSIBLE MECHANISMS OF HEARING LOSS IN AD PATIENTS
ARHL is a complex phenomenon caused by multiple risk factors. In most cases, patients experience functional changes in both the peripheral and central auditory systems. On the other hand, hearing loss in the peripheral only will also result in alteration in the central auditory pathway later. In AD-related ARHL, similar circumstances are also observed in the clinic. Therefore, here we discuss the possible causes of ARHL in AD patients, focusing on the roles of Aβ and Tau within both the peripheral and central auditory systems [Figure 2].
Figure 2. Schematic diagram showing the impacts of Aβ deposition in the brain and peripheral organs. In the central nervous system, abnormal deposition of Aβ in the brain leads to mitochondria dysfunction, which then further causes synaptic degeneration to induce changes in the auditory pathway. Aβ could also exert influences on peripheral organs either directly or indirectly through lymphatic fluid with inflammatory factors, such as IL-1β and TNF-α released by activated microglia cells. Aβ: Beta-amyloid.
Pathogenesis factors
The direct effect of β-amyloid and tau deposition in the inner ear
In the previous section, we have discussed the deposition of Aβ and tau in the CNS and its related neurotoxicity. In fact, with the comprehensive investigation of AD pathology, it has been discovered that there is a specific deposition of Aβ and tau protein in the peripheral region of AD patients, which exerts detrimental effects on the functionality of associated organs.
Retinal abnormalities in vascular structure and Aβ accumulation have been identified in individuals with AD[99,100]. Shi et al. examined postmortem retinas from 56 human donors and observed an early and progressive decline in vascular PDGFRβ among patients with mild cognitive impairment (MCI) and AD, while retinal loss of PDGFRβ was significantly linked to elevated levels of retinal vascular Aβ40 and Aβ42[101]. Additionally, deposition of Aβ has been detected within the gastrointestinal tract both in individuals diagnosed with AD and transgenic mice overexpressing APP[102]. Similarly, previous studies have demonstrated the deposition of hyperphosphorylated tau (p-tau) in retinal ganglion cells (RGC) of AD patients with visual abnormalities[103].
These findings in Aβ-related pathology in the retina and gastrointestinal tract could provide valuable insights into cochlear-related changes. Notably, Aβ and tau levels exhibit a strong correlation with the severity of hearing impairment. In a recent cross-sectional study[104], a significant association was found between ARHL and Aβ, as well as tau levels measured via PET scans. Certain animal studies[105] have generated genetically modified mice that specifically express Aβ in their cochleae. These mice exhibited impaired perception of high-frequency sound due to Aβ expression within their cochlear hair cells while experiencing hair cell loss at the basal turn. However, these studies solely observed peripheral Aβ deposition, and the mechanisms by which Aβ reaches these sites, whether it is locally synthesized or transported from the brain to the periphery, remain unknown. Further investigations are warranted.
The possibility of flow from the CSF to the endolymphatic fluid of the cochlea
In light of the communication between CSF and inner ear fluid, a study[106] has demonstrated that AAV-Slc17a8 injected into the CSF effectively restored auditory function in a mouse model of hereditary deafness. This study characterized the transport of AAV-Slc17a8 through the cochlear aqueduct. However, there is currently no definitive research conclusion regarding the deposition of Aβ in the cochlea. Additionally, due to the small volume of cochlear endolymphatic fluid, manual removal presents technical challenges. Nevertheless, considering the connection between CSF and inner ear lymph fluid[106], an alternative approach for investigating early hearing loss in AD patients involves injecting Aβ and tracers into the brain to determine if they can reach the endolymphatic fluid within the cochlea. Assuming their presence, neurotoxic Aβ may directly impact various components such as blood-ear barrier integrity, hair cells, and spiral ganglia. Even without direct deposition of Aβ in these areas, Fukuda et al. explored how cerebral amyloidosis affects cochlear properties and revealed that the total protein content surrounding lymph in AD model mice was approximately 1.5 times higher than that observed in WT mice[107]. This difference may be attributed to significant changes in proteins associated with cellular damage and inflammatory response[108]. Consequently, peri-lymphoid homeostasis is disrupted.
Central auditory pathway dysfunction
With the aging process, there is a decline in the auditory system, which is not only influenced by the loss of peripheral hair cells and dysfunction of stria vascularis but also by corresponding changes in the central auditory system. Calcium-binding proteins (CBPs), namely parvalbumin (PV) and calreticulin (CR), serve as major fast cytoplasmic calcium buffers in the central nervous system, safeguarding neurons against damage caused by elevated intracellular Ca2+[109]. A study investigating age-related alterations in the central auditory system of rats[110] discovered a significant decrease in both the number and average volume of calcium-binding protein immunoreactive (CB-IR) cells within the ventral region of the medial geniculate body (MGB) with advancing age, showing a significant correlation between CBP levels and age. These observed age-related changes in CR may contribute to deterioration in central age-related hearing function. Furthermore, researchers found an age-associated reduction in glycine levels within the cochlear nucleus (CN) region in rats[111]. In humans, aging leads to cortical atrophy accompanied by reductions in gray matter (GM) and white matter (WM) volumes, along with an expansion of CSF space[112,113]. Guo et al. discovered that older women with cortical atrophy exhibited more severe hearing impairment compared to women without cortical atrophy, and this association was particularly pronounced in the left ear[114]. Glutamate serves as the primary excitatory neurotransmitter in the brain, and numerous studies have reported age-related reductions in various brain regions, such as the motor cortex, parietal cortex, basal ganglia[115], hippocampus, and anterior cingulate cortex (ACC)[116]. It is widely recognized that multiple forms of Aβ deposition contribute to abnormal tau aggregation, synaptic dysfunction, cell death, and a hierarchical cascade of brain atrophy[117,118]. Studies conducted on 12-month-old transgenic mice expressing APP[119] revealed a significant increase in the number of mitochondria, accompanied by a notable decrease in their length. Additionally, levels of mitochondrial fission protein (Drp1) were found to be elevated, while synaptic and dendritic protein levels exhibited a decline. Furthermore, a significant reduction was observed in dendritic spines. Consequently, it can be inferred that the presence of Aβ deposition is responsible for the mitochondrial dynamic abnormalities and biogenetic defects, as well as the alterations in both structure and function within the mitochondria of APP mice. Aβ deposition exerts similar influences on the central auditory pathway. In 5xFAD mice, central auditory processing disorders (CAPDs) and hearing loss were found to be associated with AD and may precede the onset of AD[120]. It is possible that the abnormal deposition of Aβ leads to deterioration of the sensory system integrity by reducing the number of neuronal populations actively engaged in auditory information processing or deteriorating synchronously firing properties of neurons, as demonstrated in Hidisoglu and Yargicoglu[121]. In their study, administration of Aβ1-42 peptide in the lateral ventricles in rats causes significant changes in auditory evoked potentials. Additionally, Aβ deposition was observed in various regions of the mouse brain, including auditory cortex (AC), insular cortex (IC), and MGB. This distribution pattern resembles histological findings observed in AD patients[122] and is linked to age-related CAPDs.
Influence of decreased auditory inputs on cognition
Several studies have demonstrated a significant association between reduced auditory inputs caused by ARHL and cognitive impairment, as well as an elevated risk of developing AD. Currently, the prevailing hypothesis can be broadly categorized into three main aspects[123].
The common cause theory
ARHL and cognitive impairment may stem from shared risk factors. As individuals age, systemic aging processes in the body contribute to widespread brain atrophy, metabolic decline, oxidative damage, neuroinflammation, and cardiovascular diseases. These factors collectively exert detrimental effects both on the brain and auditory periphery.
Social isolation and depression
This is particularly relevant for patients with ARHL, who may gradually withdraw from social activities due to communication challenges, leading to feelings of loneliness and depression. Insufficient social interaction is recognized as detrimental to mental health, and it is well-established that depression can exacerbate cognitive decline. Approximately two-thirds of individuals aged 70 and older experience hearing loss. These patients often encounter feelings of frustration or embarrassment stemming from their communication difficulties, which may prompt them to disengage from social gatherings, thereby fostering social isolation and loneliness. Research indicates that the sense of loneliness among elderly individuals with hearing impairments is 2.2 times greater than that experienced by their counterparts without such impairments, while the likelihood of social isolation increases by a factor of 1.52 for every additional 10 dB increase in hearing loss[124]. Prolonged periods of social isolation are associated with elevated levels of stress hormones (such as cortisol), which can adversely affect brain structure and function, consequently heightening the risk of developing depression.
Cognitive load increase
Within a given period, the amount of information that the brain can process, store, and access is limited. The long-term hearing issues of patients with ARHL will cause the brain to consume more energy in processing sound information, thereby reducing the energy allocation available for other cognitive tasks such as memory. As time progresses, this continuous cognitive burden might lead to alterations in the structure and function of the brain, thereby influencing overall cognitive health. The temporal lobe is situated on both sides of the brain, near the ears, and plays a key role in numerous significant cognitive and sensory functions. In an MRI study[125] of elderly individuals without a history of neurosurgery, severe heart disease, lung disease, or metastatic cancer, peripheral hearing loss was independently associated with an accelerated decline in total brain volume and atrophy of the temporal lobe. Some longitudinal studies have indicated that ARHL might precede the onset of clinical dementia by 5-15 years[126], and for every 10 dB increase in hearing loss above 25 dB, the risk of developing dementia increases by 20%[127]. The dorsolateral prefrontal cortex (DLPFC), an important area of the frontal lobe of the brain, plays a vital role in multiple advanced cognitive functions[128]. Research has discovered that in patients with ARHL, the distal functional coupling between the DLPFC and the AC significantly increases, and the higher the degree of hearing loss, the stronger the coupling. Likewise, the hippocampus, as one of the important structures of the frontal lobe, has two renowned functions - supporting episodic memory and spatial navigation. Research has found that listeners with hearing loss are less sensitive to spatial cues, worsening performance on the selective attention task[129]. Moreover, with the advancement of research, the functional theory of the hippocampus might have long surpassed these. Auditory stimulation can regulate the rhythm of the hippocampus, holding significance for enhancing memory and alleviating cognitive decline[130,131]. Studies on humans and non-human primates have revealed that there exists a common effective connectivity signal that directly connects the AC to the ventrolateral prefrontal cortex (VLPFC) and indirectly projects to the hippocampus[132]. The tendency of energy allocation is important for the metabolic balance of the brain. In particular, gamma-aminobutyric acid (GABA), the principal inhibitory neurotransmitter in the central nervous system, is of great significance for maintaining the balance and function of the nervous system. The secretion of GABA in the ACC, which controls behavior under high cognitive load conditions, increases[133]. However, GABA in the temporal lobe auditory center of ARHL patients decreases[134], and increasing the GABA or the sensitivity of its receptors in the AC can enhance the response of the auditory center to sound stimuli[135].
CONCLUSION
In conclusion, the accumulating evidence highlights the importance of hearing loss in relation to aging and neurodegeneration. The impact of varying degrees of hearing loss on AD is varied. Specifically, there is a clear upward trend in the risk of developing AD as the severity of hearing loss increases. This observation strongly suggests a potential dose-response relationship between the degree of hearing loss and AD, offering a novel perspective for understanding the interaction between these two conditions. This review is based on the in-depth discussion regarding the correlation between ARHL and AD, demonstrating significant practical implications and potential applications. Primarily, by elucidating the intricate relationship between these two conditions, it offers novel perspectives and insights for clinicians. In the process of diagnosing and treating elderly patients with multiple intertwined diseases, healthcare professionals often encounter complex scenarios. Thus, recognizing the association between hearing loss and Alzheimer’s disease can serve as a crucial starting point. Nevertheless, there are still a few critical links between ARHL and AD that remain unresolved. For instance, (1) which condition occurs first, ARHL or AD? Or do they both result from a common underlying mechanism? (2) How does the deposition of Aβ and tau proteins in the inner ear take place? and (3) What molecular mechanisms drive AD-related ARHL? Understanding these connections enables researchers to comprehensively assess patients’ overall health status while further developing precise diagnosis and treatment plans.
DECLARATIONS
Authors’ contributions
Participated in literature search, writing, and original draft preparation: Ma Y, Yang Y, Zhou Y
Conceptualization, review, revision, and editing: Chai R, Le W, Ma Y
Availability of data and materials
Not applicable.
Financial support and sponsorship
This study was supported by the National Natural Science Foundation of China (Project No. 82201302) and the National Key R&D Program of China (Project No. 2023YFC2509800).
Conflicts of interest
Le W is the Editor-in-Chief of Ageing and Neurodegenerative Diseases. However, he was not involved in any steps during the editorial handling of the manuscript, inviting Reviewers, or making editorial decisions regarding this submission. The other authors declared that there are no conflicts of interest.
Ethical approval and consent to participate
Not applicable.
Consent for publication
Not applicable.
Copyright
© The Author(s) 2024.
REFERENCES
2. Paciello F, Pisani A, Rinaudo M, et al. Noise-induced auditory damage affects hippocampus causing memory deficits in a model of early age-related hearing loss. Neurobiol Dis 2023;178:106024.
3. Lin BM, Wang M, Stankovic KM, et al. Cigarette smoking, smoking cessation, and risk of hearing loss in women. Am J Med 2020;133:1180-6.
4. Kros CJ, Steyger PS. Aminoglycoside- and cisplatin-induced ototoxicity: mechanisms and otoprotective strategies. Cold Spring Harb Perspect Med 2019;9:a033548.
5. Shen Y, Ye B, Chen P, et al. Cognitive decline, dementia, Alzheimer’s disease and presbycusis: examination of the possible molecular mechanism. Front Neurosci 2018;12:394.
6. Jafari Z, Kolb BE, Mohajerani MH. Age-related hearing loss and tinnitus, dementia risk, and auditory amplification outcomes. Ageing Res Rev 2019;56:100963.
7. Chern A, Golub JS. Age-related hearing loss and dementia. Alzheimer Dis Assoc Disord 2019;33:285-90.
8. He P, Wen X, Hu X, et al. Hearing aid acquisition in chinese older adults with hearing loss. Am J Public Health 2018;108:241-7.
12. Sheladia S, Reddy PH. Age-related chronic diseases and Alzheimer’s disease in texas: a hispanic focused study. J Alzheimers Dis Rep 2021;5:121-33.
13. Gowda P, Reddy PH, Kumar S. Deregulated mitochondrial microRNAs in Alzheimer’s disease: focus on synapse and mitochondria. Ageing Res Rev 2022;73:101529.
16. Power R, Prado-Cabrero A, Mulcahy R, Howard A, Nolan JM. The role of nutrition for the aging population: implications for cognition and Alzheimer’s disease. Annu Rev Food Sci Technol 2019;10:619-39.
17. Kay DW, Roth M, Beamish P. Old age mental disorders in newcastle upon tyen. II. A study of possible social and medical causes. Br J Psychiatry 1964;110:668-82.
18. Kiely KM, Gopinath B, Mitchell P, Luszcz M, Anstey KJ. Cognitive, health, and sociodemographic predictors of longitudinal decline in hearing acuity among older adults. J Gerontol A Biol Sci Med Sci 2012;67:997-1003.
19. Rutherford BR, Brewster K, Golub JS, Kim AH, Roose SP. Sensation and psychiatry: linking age-related hearing loss to late-life depression and cognitive decline. Am J Psychiatry 2018;175:215-24.
20. Stickel AM, Mendoza A, Tarraf W, et al. Hearing loss and associated 7-year cognitive outcomes among hispanic and latino adults. JAMA Otolaryngol Head Neck Surg 2024;150:385-92.
21. Cantuaria ML, Pedersen ER, Waldorff FB, et al. Hearing loss, hearing aid use, and risk of dementia in older adults. JAMA Otolaryngol Head Neck Surg 2024;150:157-64.
22. Lin FR, Pike JR, Albert MS, et al; ACHIEVE Collaborative Research Group. Hearing intervention versus health education control to reduce cognitive decline in older adults with hearing loss in the USA (ACHIEVE): a multicentre, randomised controlled trial. Lancet 2023;402:786-97.
23. Kwok SS, Nguyen XT, Wu DD, Mudar RA, Llano DA. Pure tone audiometry and hearing loss in Alzheimer’s disease: a meta-analysis. Front Psychol 2021;12:788045.
24. Johnson JCS, Marshall CR, Weil RS, Bamiou DE, Hardy CJD, Warren JD. Hearing and dementia: from ears to brain. Brain 2021;144:391-401.
25. Merchant SN, Nadol Jr JB. Schuknecht’s pathology of the ear. 3rd ed. Shelton, CT: People’s Medical House-USA; 2010. Available from: https://books.google.com/books?id=cUEKaR0x-XUC&printsec=frontcover&hl=zh-CN#v=onepage&q&f=false. [Last accessed on 27 Nov 2024].
26. Schuknecht HF, Gacek MR. Cochlear pathology in presbycusis. Ann Otol Rhinol Laryngol 1993;102:1-16.
27. Suga F, Lindsay JR. Histopathological observations of presbycusis. Ann Otol Rhinol Laryngol 1976;85:169-84.
28. Ohlemiller KK. Age-related hearing loss: the status of Schuknecht’s typology. Curr Opin Otolaryngol Head Neck Surg 2004;12:439-43.
29. Bhattacharyya TK, Dayal VS. Influence of age on hair cell loss in the rabbit cochlea. Hear Res 1989;40:179-83.
30. Wright A, Davis A, Bredberg G, Ulehlova L, Spencer H. Hair cell distributions in the normal human cochlea. Acta Otolaryngol Suppl 1987;444:1-48.
31. Makary CA, Shin J, Kujawa SG, Liberman MC, Merchant SN. Age-related primary cochlear neuronal degeneration in human temporal bones. J Assoc Res Otolaryngol 2011;12:711-7.
32. Pauler M, Schuknecht HF, Thornton AR. Correlative studies of cochlear neuronal loss with speech discrimination and pure-tone thresholds. Arch Otorhinolaryngol 1986;243:200-6.
33. Pauler M, Schuknecht HF, White JA. Atrophy of the stria vascularis as a cause of sensorineural hearing loss. Laryngoscope 1988;98:754-9.
34. Schuknecht HF. Further observations on the pathology of presbycusis. Arch Otolaryngol 1964;80:369-82.
35. Nelson EG, Hinojosa R. Presbycusis: a human temporal bone study of individuals with downward sloping audiometric patterns of hearing loss and review of the literature. Laryngoscope 2006;116:1-12.
36. Yamasoba T, Lin FR, Someya S, Kashio A, Sakamoto T, Kondo K. Current concepts in age-related hearing loss: epidemiology and mechanistic pathways. Hear Res 2013;303:30-8.
38. Böttger EC, Schacht J. The mitochondrion: a perpetrator of acquired hearing loss. Hear Res 2013;303:12-9.
39. Someya S, Prolla TA. Mitochondrial oxidative damage and apoptosis in age-related hearing loss. Mech Ageing Dev 2010;131:480-6.
40. Darrat I, Ahmad N, Seidman K, Seidman MD. Auditory research involving antioxidants. Curr Opin Otolaryngol Head Neck Surg 2007;15:358-63.
41. Seidman MD. Effects of dietary restriction and antioxidants on presbyacusis. Laryngoscope 2000;110:727-38.
42. Yamasoba T, Someya S, Yamada C, Weindruch R, Prolla TA, Tanokura M. Role of mitochondrial dysfunction and mitochondrial DNA mutations in age-related hearing loss. Hear Res 2007;226:185-93.
43. Jiang H, Talaska AE, Schacht J, Sha SH. Oxidative imbalance in the aging inner ear. Neurobiol Aging 2007;28:1605-12.
44. Coling D, Chen S, Chi LH, Jamesdaniel S, Henderson D. Age-related changes in antioxidant enzymes related to hydrogen peroxide metabolism in rat inner ear. Neurosci Lett 2009;464:22-5.
45. Mikhed Y, Daiber A, Steven S. Mitochondrial oxidative stress, mitochondrial DNA damage and their role in age-related vascular dysfunction. Int J Mol Sci 2015;16:15918-53.
47. Tracy RP. Emerging relationships of inflammation, cardiovascular disease and chronic diseases of aging. Int J Obes Relat Metab Disord 2003;27 Suppl 3:S29-34.
48. Adams JC. Clinical implications of inflammatory cytokines in the cochlea: a technical note. Otol Neurotol 2002;23:316-22.
49. Wells HRR, Newman TA, Williams FMK. Genetics of age-related hearing loss. J Neurosci Res 2020;98:1698-704.
50. Van Laer L, Huyghe JR, Hannula S, et al. A genome-wide association study for age-related hearing impairment in the Saami. Eur J Hum Genet 2010;18:685-93.
51. Arsenijevic D, Onuma H, Pecqueur C, et al. Disruption of the uncoupling protein-2 gene in mice reveals a role in immunity and reactive oxygen species production. Nat Genet 2000;26:435-9.
52. Unal M, Tamer L, Doğruer ZN, Yildirim H, Vayisoğlu Y, Camdeviren H. N-acetyltransferase 2 gene polymorphism and presbycusis. Laryngoscope 2005;115:2238-41.
53. Boucher S, Tai FWJ, Delmaghani S, et al. Ultrarare heterozygous pathogenic variants of genes causing dominant forms of early-onset deafness underlie severe presbycusis. Proc Natl Acad Sci U S A 2020;117:31278-89.
54. Girotto G, Pirastu N, Sorice R, et al. Hearing function and thresholds: a genome-wide association study in European isolated populations identifies new loci and pathways. J Med Genet 2011;48:369-74.
55. Ozben T, Ozben S. Neuro-inflammation and anti-inflammatory treatment options for Alzheimer’s disease. Clin Biochem 2019;72:87-9.
56. Liu T, Woo JA, Yan Y, LePochat P, Bukhari MZ, Kang DE. Dual role of cofilin in APP trafficking and amyloid-β clearance. FASEB J 2019;33:14234-47.
57. Blennow K, Zetterberg H. Biomarkers for Alzheimer’s disease: current status and prospects for the future. J Intern Med 2018;284:643-63.
58. Hansson O, Lehmann S, Otto M, Zetterberg H, Lewczuk P. Advantages and disadvantages of the use of the CSF Amyloid β (Aβ) 42/40 ratio in the diagnosis of Alzheimer’s disease. Alzheimers Res Ther 2019;11:34.
59. Blennow K. A review of fluid biomarkers for Alzheimer’s disease: moving from CSF to blood. Neurol Ther 2017;6:15-24.
60. Vaillant-Beuchot L, Mary A, Pardossi-Piquard R, et al. Accumulation of amyloid precursor protein C-terminal fragments triggers mitochondrial structure, function, and mitophagy defects in Alzheimer’s disease models and human brains. Acta Neuropathol 2021;141:39-65.
61. Nabers A, Perna L, Lange J, et al. Amyloid blood biomarker detects Alzheimer’s disease. EMBO Mol Med 2018;10:e8763.
62. John A, Reddy PH. Synaptic basis of Alzheimer’s disease: focus on synaptic amyloid beta, P-tau and mitochondria. Ageing Res Rev 2021;65:101208.
63. Zhu M, Wang X, Sun L, Schultzberg M, Hjorth E. Can inflammation be resolved in Alzheimer’s disease? Ther Adv Neurol Disord 2018;11:1756286418791107.
64. Blasko I, Veerhuis R, Stampfer-Kountchev M, Saurwein-Teissl M, Eikelenboom P, Grubeck-Loebenstein B. Costimulatory effects of interferon-gamma and interleukin-1beta or tumor necrosis factor alpha on the synthesis of Abeta1-40 and Abeta1-42 by human astrocytes. Neurobiol Dis 2000;7:682-9.
65. Kerr JS, Adriaanse BA, Greig NH, et al. Mitophagy and Alzheimer’s disease: cellular and molecular mechanisms. Trends Neurosci 2017;40:151-66.
66. Swerdlow RH. Mitochondria and mitochondrial cascades in Alzheimer’s disease. J Alzheimers Dis 2018;62:1403-16.
67. Liang T, Hang W, Chen J, et al. ApoE4 (Δ272-299) induces mitochondrial-associated membrane formation and mitochondrial impairment by enhancing GRP75-modulated mitochondrial calcium overload in neuron. Cell Biosci 2021;11:50.
69. Song M, Fan X. Systemic metabolism and mitochondria in the mechanism of Alzheimer’s disease: finding potential therapeutic targets. Int J Mol Sci 2023;24:8398.
70. Shoshan-Barmatz V, Nahon-Crystal E, Shteinfer-Kuzmine A, Gupta R. VDAC1, mitochondrial dysfunction, and Alzheimer’s disease. Pharmacol Res 2018;131:87-101.
71. Olesen MA, Villavicencio-Tejo F, Quintanilla RA. The use of fibroblasts as a valuable strategy for studying mitochondrial impairment in neurological disorders. Transl Neurodegener 2022;11:36.
72. James D, Kang S, Park S. Injection of β-amyloid into the hippocampus induces metabolic disturbances and involuntary weight loss which may be early indicators of Alzheimer’s disease. Aging Clin Exp Res 2014;26:93-8.
73. Liguori C, Chiaravalloti A, Sancesario G, et al. Cerebrospinal fluid lactate levels and brain [18F]FDG PET hypometabolism within the default mode network in Alzheimer’s disease. Eur J Nucl Med Mol Imaging 2016;43:2040-9.
74. Bhargava P, Schnellmann RG. Mitochondrial energetics in the kidney. Nat Rev Nephrol 2017;13:629-46.
75. Tönnies E, Trushina E. Oxidative stress, synaptic dysfunction, and Alzheimer’s disease. J Alzheimers Dis 2017;57:1105-21.
77. Guan S, Zhao L, Peng R. Mitochondrial respiratory chain supercomplexes: from structure to function. Int J Mol Sci 2022;23:13880.
78. Liu Y, Huang Y, Xu C, et al. Mitochondrial dysfunction and therapeutic perspectives in cardiovascular diseases. Int J Mol Sci 2022;23:16053.
79. Brand MD. Mitochondrial generation of superoxide and hydrogen peroxide as the source of mitochondrial redox signaling. Free Radic Biol Med 2016;100:14-31.
80. Moreira PI, Carvalho C, Zhu X, Smith MA, Perry G. Mitochondrial dysfunction is a trigger of Alzheimer’s disease pathophysiology. Biochim Biophys Acta 2010;1802:2-10.
81. Mi Y, Qi G, Brinton RD, Yin F. Mitochondria-targeted therapeutics for Alzheimer’s disease: the good, the bad, the potential. Antioxid Redox Signal 2021;34:611-30.
82. Shimada K, Crother TR, Karlin J, et al. Oxidized mitochondrial DNA activates the NLRP3 inflammasome during apoptosis. Immunity 2012;36:401-14.
83. Zhao M, Wang Y, Li L, et al. Mitochondrial ROS promote mitochondrial dysfunction and inflammation in ischemic acute kidney injury by disrupting TFAM-mediated mtDNA maintenance. Theranostics 2021;11:1845-63.
84. O’Hara R, Tedone E, Ludlow A, et al. Quantitative mitochondrial DNA copy number determination using droplet digital PCR with single-cell resolution. Genome Res 2019;29:1878-88.
85. Nikolac Perkovic M, Videtic Paska A, Konjevod M, et al. Epigenetics of Alzheimer’s disease. Biomolecules 2021;11:195.
86. Li Y, Xia X, Wang Y, Zheng JC. Mitochondrial dysfunction in microglia: a novel perspective for pathogenesis of Alzheimer’s disease. J Neuroinflammation 2022;19:248.
87. Podlesniy P, Figueiro-Silva J, Llado A, et al. Low cerebrospinal fluid concentration of mitochondrial DNA in preclinical Alzheimer disease. Ann Neurol 2013;74:655-68.
88. Crary JF, Trojanowski JQ, Schneider JA, et al. Primary age-related tauopathy (PART): a common pathology associated with human aging. Acta Neuropathol 2014;128:755-66.
89. Zhu B, Liu Y, Hwang S, et al. Trem2 deletion enhances tau dispersion and pathology through microglia exosomes. Mol Neurodegener 2022;17:58.
92. Shin MK, Vázquez-Rosa E, Koh Y, et al. Reducing acetylated tau is neuroprotective in brain injury. Cell 2021;184:2715-32.e23.
93. Liu CC, Liu CC, Kanekiyo T, Xu H, Bu G. Apolipoprotein E and Alzheimer disease: risk, mechanisms and therapy. Nat Rev Neurol 2013;9:106-18.
94. Huang YA, Zhou B, Wernig M, Südhof TC. ApoE2, ApoE3, and ApoE4 differentially stimulate APP transcription and Aβ secretion. Cell 2017;168:427-41.e21.
95. Robert J, Button EB, Yuen B, et al. Clearance of beta-amyloid is facilitated by apolipoprotein E and circulating high-density lipoproteins in bioengineered human vessels. Elife 2017;6:e29595.
96. Hashimoto T, Serrano-Pozo A, Hori Y, et al. Apolipoprotein E, especially apolipoprotein E4, increases the oligomerization of amyloid β peptide. J Neurosci 2012;32:15181-92.
97. Bonham LW, Desikan RS, Yokoyama JS; Alzheimer’s disease neuroimaging initiative. The relationship between complement factor C3, APOE ε4, amyloid and tau in Alzheimer’s disease. Acta Neuropathol Commun 2016;4:65.
98. Dorey E, Bamji-Mirza M, Najem D, et al. Apolipoprotein E isoforms differentially regulate Alzheimer’s disease and amyloid-β-induced inflammatory response in vivo and in vitro. J Alzheimers Dis 2017;57:1265-79.
99. den Haan J, Morrema THJ, Verbraak FD, et al. Amyloid-beta and phosphorylated tau in post-mortem Alzheimer’s disease retinas. Acta Neuropathol Commun 2018;6:147.
100. Doustar J, Torbati T, Black KL, Koronyo Y, Koronyo-Hamaoui M. Optical coherence tomography in Alzheimer’s disease and other neurodegenerative diseases. Front Neurol 2017;8:701.
101. Shi H, Koronyo Y, Rentsendorj A, et al. Identification of early pericyte loss and vascular amyloidosis in Alzheimer’s disease retina. Acta Neuropathol 2020;139:813-36.
102. Eisele YS, Duyckaerts C. Propagation of Aß pathology: hypotheses, discoveries, and yet unresolved questions from experimental and human brain studies. Acta Neuropathol 2016;131:5-25.
103. Gaire BP, Koronyo Y, Fuchs DT, et al. Alzheimer’s disease pathophysiology in the Retina. Prog Retin Eye Res 2024;101:101273.
104. Zheng M, Yan J, Hao W, et al. Worsening hearing was associated with higher β-amyloid and tau burden in age-related hearing loss. Sci Rep 2022;12:10493.
105. Omata Y, Tharasegaran S, Lim YM, et al. Expression of amyloid-β in mouse cochlear hair cells causes an early-onset auditory defect in high-frequency sound perception. Aging 2016;8:427-39.
106. Mathiesen BK, Miyakoshi LM, Cederroth CR, et al. Delivery of gene therapy through a cerebrospinal fluid conduit to rescue hearing in adult mice. Sci Transl Med 2023;15:eabq3916.
107. Fukuda M, Okanishi H, Ino D, et al. Disturbance in the protein landscape of cochlear perilymph in an Alzheimer’s disease mouse model. PLoS One 2024;19:e0303375.
108. Saito T, Matsuba Y, Mihira N, et al. Single App knock-in mouse models of Alzheimer’s disease. Nat Neurosci 2014;17:661-3.
109. Toescu EC, Verkhratsky A, Landfield PW. Ca2+ regulation and gene expression in normal brain aging. Trends Neurosci 2004;27:614-20.
110. Ouda L, Burianova J, Syka J. Age-related changes in calbindin and calretinin immunoreactivity in the central auditory system of the rat. Exp Gerontol 2012;47:497-506.
111. Banay-Schwartz M, Lajtha A, Palkovits M. Changes with aging in the levels of amino acids in rat CNS structural elements. II. Taurine and small neutral amino acids. Neurochem Res 1989;14:563-70.
112. Courchesne E, Chisum HJ, Townsend J, et al. Normal brain development and aging: quantitative analysis at in vivo MR imaging in healthy volunteers. Radiology 2000;216:672-82.
113. Lemaitre H, Goldman AL, Sambataro F, et al. Normal age-related brain morphometric changes: nonuniformity across cortical thickness, surface area and gray matter volume? Neurobiol Aging 2012;33:617.e1-9.
114. Guo X, Skoog I, Idrizbegovic E, Pantoni L, Simoni M, Rosenhall U. Hearing loss and cortical atrophy in a population-based study on non-demented women. Age Ageing 2008;37:333-6.
115. Kaiser LG, Schuff N, Cashdollar N, Weiner MW. Age-related glutamate and glutamine concentration changes in normal human brain: 1H MR spectroscopy study at 4 T. Neurobiol Aging 2005;26:665-72.
116. Schubert F, Gallinat J, Seifert F, Rinneberg H. Glutamate concentrations in human brain using single voxel proton magnetic resonance spectroscopy at 3 Tesla. Neuroimage 2004;21:1762-71.
117. Jack CR Jr, Knopman DS, Jagust WJ, et al. Hypothetical model of dynamic biomarkers of the Alzheimer’s pathological cascade. Lancet Neurol 2010;9:119-28.
118. Li K, Qu H, Ma M, et al. Correlation between brain structure atrophy and plasma amyloid-β and phosphorylated tau in patients with Alzheimer’s disease and amnestic mild cognitive impairment explored by surface-based morphometry. Front Aging Neurosci 2022;14:816043.
119. Reddy PH, Yin X, Manczak M, et al. Mutant APP and amyloid beta-induced defective autophagy, mitophagy, mitochondrial structural and functional changes and synaptic damage in hippocampal neurons from Alzheimer’s disease. Hum Mol Genet 2018;27:2502-16.
120. Na D, Zhang J, Beaulac HJ, et al. Increased central auditory gain in 5xFAD Alzheimer’s disease mice as an early biomarker candidate for Alzheimer’s disease diagnosis. Front Neurosci 2023;17:1106570.
121. Hidisoglu E, Yargicoglu P. Auditory evoked potentials might have the potential to serve as early indicators related to amyloid beta peptide toxicity. Adv Med Sci 2020;65:223-32.
122. Thal DR, Rüb U, Orantes M, Braak H. Phases of A beta-deposition in the human brain and its relevance for the development of AD. Neurology 2002;58:1791-800.
123. Zhao H, Wang Y, Cui L, et al. Sensorineural hearing loss and cognitive impairment: three hypotheses. Front Aging Neurosci 2024;16:1368232.
124. Shukla A, Harper M, Pedersen E, et al. Hearing loss, loneliness, and social isolation: a systematic review. Otolaryngol Head Neck Surg 2020;162:622-33.
125. Qian ZJ, Chang PD, Moonis G, Lalwani AK. A novel method of quantifying brain atrophy associated with age-related hearing loss. Neuroimage Clin 2017;16:205-9.
126. Albers MW, Gilmore GC, Kaye J, et al. At the interface of sensory and motor dysfunctions and Alzheimer’s disease. Alzheimers Dement 2015;11:70-98.
127. Lin FR, Metter EJ, O’Brien RJ, Resnick SM, Zonderman AB, Ferrucci L. Hearing loss and incident dementia. Arch Neurol 2011;68:214-20.
128. Luan Y, Wang C, Jiao Y, Tang T, Zhang J, Teng GJ. Prefrontal-temporal pathway mediates the cross-modal and cognitive reorganization in sensorineural hearing loss with or without tinnitus: a multimodal MRI study. Front Neurosci 2019;13:222.
129. Dai L, Best V, Shinn-Cunningham BG. Sensorineural hearing loss degrades behavioral and physiological measures of human spatial selective auditory attention. Proc Natl Acad Sci U S A 2018;115:E3286-95.
130. Martorell AJ, Paulson AL, Suk HJ, et al. Multi-sensory gamma stimulation ameliorates Alzheimer’s-associated pathology and improves cognition. Cell 2019;177:256-71.e22.
131. Harrington MO, Cairney SA. Sounding it out: auditory stimulation and overnight memory processing. Curr Sleep Med Rep 2021;7:112-9.
132. Rocchi F, Oya H, Balezeau F, et al. Common fronto-temporal effective connectivity in humans and monkeys. Neuron 2021;109:852-68.e8.
133. Vassena E, Deraeve J, Alexander WH. Surprise, value and control in anterior cingulate cortex during speeded decision-making. Nat Hum Behav 2020;4:412-22.
134. Zemaitis K, Kaliyappan K, Frerichs V, Friedman A, Krishnan Muthaiah VP. Mass spectrometry imaging of blast overpressure induced modulation of GABA/glutamate levels in the central auditory neuraxis of Chinchilla. Exp Mol Pathol 2021;119:104605.
Cite This Article

How to Cite
Ma, Y.; Yang, Y.; Zhou, Y.; Le, W.; Chai, R. Age-related hearing loss: possible associations with Alzheimer's disease. Ageing. Neur. Dis. 2024, 4, 17. http://dx.doi.org/10.20517/and.2024.24
Download Citation
Export Citation File:
Type of Import
Tips on Downloading Citation
Citation Manager File Format
Type of Import
Direct Import: When the Direct Import option is selected (the default state), a dialogue box will give you the option to Save or Open the downloaded citation data. Choosing Open will either launch your citation manager or give you a choice of applications with which to use the metadata. The Save option saves the file locally for later use.
Indirect Import: When the Indirect Import option is selected, the metadata is displayed and may be copied and pasted as needed.
About This Article
Copyright
Data & Comments
Data
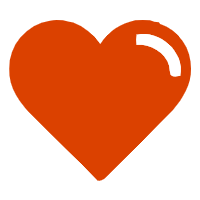
Comments
Comments must be written in English. Spam, offensive content, impersonation, and private information will not be permitted. If any comment is reported and identified as inappropriate content by OAE staff, the comment will be removed without notice. If you have any queries or need any help, please contact us at support@oaepublish.com.